An entirely different technique to determine position and velocity was brought to bear in the spacecraft which relied on sightings of the stars, Earth and the Moon. It was designed by MIT under the direction of Charles Stark Draper. To reinforce his faith that his team could successfully come up with an accurate system to navigate to the Moon and back, and somewhat to the mirth of folks at NASA, he put himself forward as an astronaut candidate. The MIT system was based on a computer, an inertial platform, and optical devices; one of which was directly descended from an instrument used by generations of sailors to navigate across the world.
Set into the hull of the command module, opposite the hatch, were two apertures that accommodated the spacecraft’s optics. The smaller was for a so-called telescope, although it hardly justified the name as it had only a ‘times-one’ magnification. Neil Armstrong later quipped, “NASA is probably the only organisation in history that’s been sold a one-power telescope.” Its function was to give the CMP a wide-angle overview of the constellations visible at that side of the spacecraft to assist in aiming the other instrument, the sextant.
The second aperture in the hull was a disk and slit affair that accommodated the
objective optic of the sextant, a 28-power device used by the CMP to measure angles. Like a mariner’s sextant, it had two lines of sight with the ability to move one with respect to the other. The version used by marine navigators for hundreds of years works by viewing the horizon through a small telescope mounted on an arc which sweeps through one – sixth of a circle (hence the name ‘sextant’). A mirror arrangement on a radial arm permits the image of a celestial body (the Sun, Moon or a star) to be aligned with the view of the horizon. The
Apollo 16 command module Casper in lunar orbit showing the exterior apertures of the sextant and telescope. (NASA)

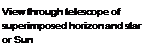



Line of to horizon
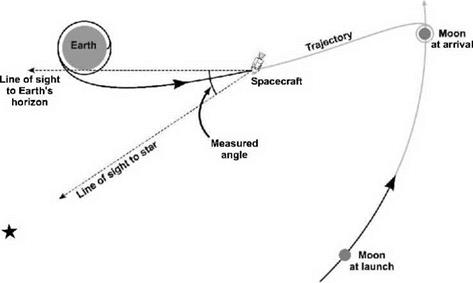
Diagram of angle measurment between planetary horizon and star.
|
angle between the two could then be read off a scale at the circumference of the arc. If carried out when the Sun was at its highest point, this measurement would yield the ship’s latitude.
The role of the sextant on the Apollo spacecraft was similarly to measure angles, and it worked in much the same way, but with major refinements. It also had two lines of sight – one fixed, the other movable – both of which peered through the slitted disk in the spacecraft’s hull. The fixed line of sight, also called the landmark line of sight (LLOS), had to be aimed by controlling the attitude of the entire spacecraft. A dense filter was placed in its light path so that the relatively bright horizons of Earth or the Moon would not swamp the stars with which they were to be compared. The movable line of sight was usually aimed at a star, and was thus called the star line of sight (SLOS). It could be swung up to 57 degrees away from the fixed line of sight to bring the image of a star into alignment with the image of the horizon. It was important that the star image be placed on that part of the horizon that was nearest to or furthest away from the star, depending on which horizon was illuminated by the Sun. Because the computer was closely integrated with the optics, the angle between the two lines of sight could be directly fed to it and used in its calculations of the state vector. The entire optical head could be rotated about the fixed line of sight and, as it did so, the disk on the outer surface of the spacecraft also turned to accommodate it. A crude sextant had been tested during the Gemini programme with mixed results. Mike Collins had tried using two hand-held models without success on Gemini 10. Later on Gemini 12, Buzz Aldrin brought one into play to help with angular measurements during a rendezvous after the spacecraft’s radar had failed.
This ability to measure the angle between a planet’s horizon and a star was what enabled onboard determination of the state vector to work. As a spacecraft coasts from one world to another, the apparent position of either orb against the stars will change, and this change will reflect the progress of the craft along its trajectory. The angle between the planet and the star at a particular time can only be valid for a single trajectory given the laws of celestial mechanics and the layout of our solar system. It can therefore be used by an onboard computer to calculate their current state vector. Repeated measurements could be used to refine the state vector. Because Program 23 in the computer was being used for this task, crews referred to their navigation task as doing a ‘P23’.
During the system’s development, experiments carried out on Gemini flights revealed difficulties of knowing exactly where Earth’s horizon was. First, having selected a star, there was a 50:50 chance that the nearest point of a planet’s horizon would be in darkness. To work around this, the CMP had to tell the computer whether he was using the nearest point or, if it was dark, the furthest point of the horizon relative to the star he was using. The second problem was that optical navigation was most sensitive when the spacecraft was near the planet on whose horizon the CMP was trying to sight. Unfortunately, the nearer they were to Earth or to the Moon, the less well-defined was the horizon. Earth’s atmosphere blurred the precise edge of its limb and the Moon’s rough terrain could make its limb decidedly knobbly when observed up close. Based on the pioneering work of Jim Lovell, who gave the onboard guidance system a workout during Apollo 8, MIT set up a simulator to train the astronauts how to choose an appropriate horizon when trying to mark on a nearby Earth or Moon.


During the flights, the CMPs made it a matter of pride to excel in their navigation exercises, even though, in most cases, their results were only meant as a backup in case communications were lost. Nevertheless, a friendly rivalry existed between some crews and the trajectory experts on Earth as to whose evaluation of the state vector was the most accurate. When Lovell put the onboard navigation system through its paces for the first time, there was a lot of interest in his results. Two days out from Earth on Apollo 8, and one day
from the Moon, Lovell informed mission control of his progress with the P23 navigation work. *Tt might be interesting to note that after sightings, we ran out P21, and we got a pericynthion of 66.8 [nautical] miles.”
What Lovell had done was to use P21 in the spacecraft’s computer. This program’s task was to determine the spacecraft’s path across a planetary surface. If the crewman entered a time, it used the current state vector to return three values; the spacecraft’s latitude and longitude directly below the ship at that time and its altitude, also at that time. As he knew roughly when they should arrive, he tried entering times at 10-minute intervals around their expected closest approach. With each advancing time entered, he noted how their predicted altitude above the lunar surface dropped, reached a minimum value, and then began to rise again. The point where it reached a minimum was their pericynthion – the spacecraft’s closest approach to the Moon. What Lovell was saying was that his predicted value for the pericynthion was very near the ideal of 60 nautical miles (110 kilometres). Bill Anders’s wit intervened. "I knew if he did it long enough, he’d finally get one that was close.”
Lovell continued to make P23 measurements and checked his resultant state vector once again with P21. Frank Borman informed Mike Collins in Houston of his results. ‘‘Mike, we ran the latest state vector we have through the P21, and it showed the pericynthion at 69.7 [nautical] miles. We’ve got the navigator, par excellence.” This may have been a gentle dig at Collins, who had been CMP on the Apollo 8 crew before standing down to undergo surgery. Nevertheless, the flight controllers were impressed. “You can tell Jim he is getting pretty ham-handed with that P21,” congratulated Collins. “He got a perilune altitude three-tenths of a mile off what we are predicting down here. Apparently, he got 69.7 [nautical miles], and the RTCC says 70.” The RTCC was the real-time computer complex, a bank of huge IBM-360 mainframe computers at mission control that were processing the radio tracking data.
Thus, at the first test of the Apollo navigation system, two entirely different systems were coming up with determinations of the spacecraft’s position that agreed to within 500 metres at a range of 300,000 kilometres out from Earth. It was a huge
confidence boost, proving that the engineers had done their work well. Procedure dictated that Lovell’s determination would be noted, but the crew would be instructed to place a switch into the correct position to accept data uplinked from the ground, w’hereby the Earth-based solution w’ould be sent up by radio and loaded directly into the onboard computer’s memory, supplanting Lovell’s effort. Apollo 8’s navigator saw the opportunity for a little one-upmanship.
“Houston, Apollo 8,’’ called Lovell.
“Apollo 8. Houston,’’ replied Collins.
Lovell then jokingly reversed the usual procedure. "Roger. If you put your [telemetry switch] to Accept, we will send you our state vector.’’ Mission control had no such switch and the request was in jest. But Lovell knew’ his state vector was as good as theirs and Collins knew’ it too. “Touche.’’ Collins responded.
Later, as Apollo 8 coasted back towards Earth, Lovell continued his P23 navigation exercises. As he did, mission control still found it hard to say w’hether his solution or the one from Earth was better. Gerry Carr informed the commander: “Frank. Let him know the state vectors have converged. They are very, very close now.”
“Is that right, Gerry?’’ replied Borman. “Okay. I’ll tell him. Thank you.”
“Don’t let his head get big. though," suggested Carr.
“You guys arc going to make it impossible to live with him.” moaned Borman. “It always was pretty hard.”
A day later. Lovell was doing even better. Carr brought the bad news. “I hate to tell you this, Frank, but that last set of marks put your state vector right on top of the [ground’s] state vector.” Borman returned with a mock plea. "Come off that, Gerry. Come on; you promised.”