In 1957, after a five-stage rocket vehicle at Wallops had reached speeds of Mach 15, PARD engineers began to study in earnest how to increase the speed of solid-propellant combinations even further. The group learned that Aerojet had developed the largest solid – propellant motor then in existence as part of its effort to convert the Jupiter to a solid-propellant missile for use aboard ship. Called the Jupiter Senior, the motor was 30 feet long and 40 inches in diameter, and it weighed 22,650 pounds, more than 3 times as much as the contemporary Sergeant missile’s motor. Using a propellant of polyurethane, ammonium perchlorate, and aluminum, the Jupiter Senior motor provided a thrust of up to about 100,000 pounds for 40 seconds in two successful static firings in March-April 1957. It eventually amassed a record of 13 static tests and 32 flights without a failure, and it prepared the way for the Aerojet motors used in Polaris and Minuteman.
About the time that the PARD engineers learned about Jupiter Senior, they found out Thiokol had discovered a way to improve the Sergeant motor by shifting from the polysulfide binder used on the missile to a polybutadiene-acrylic acid binder with metallic addi
tives. This offered a possible 20 percent increase in specific impulse. These developments led Stoney to analyze a four-stage vehicle with the Jupiter Senior as stage one, the improved Sergeant as stage two, and two Vanguard X248 motors as the third and fourth stages. Even after Sputnik, in early 1958 NACA Headquarters told the PARD team that it would not be receptive to developing a fourth launch vehicle when Vanguard, Jupiter C, and Thor-Able were well along in development or already available.33
However, with plans moving forward for what became NASA, in March 1958 NACA Headquarters asked Langley Aeronautical Laboratory to prepare a program of space technology. As a necessary part of this program, Langley included Scout—only later an acronym meaning Solid Controlled Orbital Utility Test system—to investigate human space flight and problems of reentry. The program called for $4 million to fund five vehicles for these purposes. By May 6, 1958, when Scout had become part of the space program, further analysis suggested that the third stage needed to be larger than the X248. But by then, plans for America’s space efforts were becoming so extensive that the extra costs for such development were hardly significant. By that time Langley had also arranged a contract with Thiokol for four improved Sergeant motors.34
Langley assigned Stoney as project officer but gave Thibodaux’s Rocket Section at PARD the responsibility for the initial five contracts needed to develop the Scout. A contract with Aerojet for the first stage became effective on December 1, 1958. The name of the Aerojet first stage changed from Jupiter Senior to Aerojet Senior, also called Algol I. As developed by December 1959, the motor was 29.8 feet long and 40 inches in diameter. With a steel case and polyurethane-aluminum-ammonium perchlorate propellant configured in an eight-point gear (a cylinder with eight gear-shaped, squared – off “points" radiating from it), it yielded a specific impulse of only about 215 lbf-sec/lbm and a low mass fraction of 0.838 but an average thrust of upward of 100,000 pounds depending upon the ambient temperature.35
This was a far cry from the huge boosters for the Titans and Space Shuttle, but it became the first stage of the successful Scout program. Thiokol’s second stage presented some problems. The firm was finding it difficult to adapt a new propellant for what came to be called the Castor I (or TX-33-35) second-stage motor. Initial static firings had been successful, but then Thiokol encountered unspecified difficulties that had to be overcome. Although the grain design was the same as for the Sergeant, the Castor used a polybutadiene acrylic acid-aluminum-ammonium perchlorate propel-
lant (also employed as an interim propellant on Minuteman I) and was 20.5 feet long to Sergeant’s 16.3 feet, with an identical 31-inch diameter. Once Thiokol engineers overcame developmental problems with the propellant, the Castor yielded a specific impulse of almost 275 lbf-sec/lbm to only 186 for Sergeant (although the two figures were not comparable because for Scout, the Castor was used at altitude with a larger expansion angle than for Sergeant, which launched on the ground). According to figures supplied by Thiokol, 278 Castor’s average thrust was 64,340 pounds compared to Sergeant’s Chapter 7 41,200.36
The initial four-stage Scout with all stages live flew on July 1, 1960, in the first of nine developmental flights labeled ST-1 through ST-9 (for Scout Tests 1-9), all launched from Wallops. NASA treated them as operational missions, having them carry Explorer spacecraft, ionospheric probes, and one reentry payload. The December 4, 1960, launch of ST-3 was the first attempted orbital mission with the Scout. The Algol IA first stage performed properly, but the Castor IA second stage did not ignite because of human failure to detect a defect in the ignition system. ST-4 on February 16, 1961, thus became the first entirely solid-propellant launch vehicle to achieve orbit.37
From these (by later standards) somewhat primitive beginnings, the Scout went on to incorporate more sophisticated technologies. For instance, the Antares IIA, designed by Allegany Ballistics Laboratory and produced by Hercules at its Bacchus Works in Magna, Utah, marked a considerable improvement over the first stage – three motor for the Scout, also a Hercules product. The Antares IIA featured a composite, modified, double-base propellant including ammonium perchlorate, HMX, nitrocellulose, nitroglycerin, and aluminum. Even with a smaller nozzle expansion ratio, this yielded an increase in average thrust from about 13,000 pounds for the older motor to about 21,000 for X259 Antares IIA. This stage first launched on March 29, 1962, in an early (A1) version. The A2 completed its development in June of that year, almost simultaneously with Hercules’ second stage for Polaris A2, which also used a composite-modified double-base propellant, although one without HMX and with a lower level of performance (probably reflecting the fact that the Polaris motor had an earlier date of development completion by about six months). For Polaris, HMX usage awaited the A3 version, with the Antares IIA actually using it before the missile, reversing the usual practice for launch vehicles to borrow technology from missiles.38
About this time, some earlier Scout technologies found use in other programs. For example, the thrust-augmented Thor (TAT), which entered the launch-vehicle inventory in 1963, incorporated three Thiokol TX-33-52 (Castor I) solid-propellant rocket boosters to supplement the power of the liquid-propellant first stage. The TAT consisted of a Thor with about 170,000 pounds of thrust and three Castor I solid-propellant rocket boosters, which increased liftoff thrust to 331,550 pounds.39
The TAT soon gave way to a further improved vehicle with a replacement for the Castor I. The air force’s Space Systems Division had announced contracts for a long-tank Thor (called Thorad) to replace the thrust-augmented Thor in January 1966. Douglas would provide the new Thor, with thrust augmentation continuing to be provided by Thiokol; only for the Thorad, the three solid motors would be Castor IIs. The Thorad was more than 70 feet long, as compared with 56 feet for the TAT. The added length came mainly in the form of the extended tanks that increased the burning time of the first stage. For the Castor II (TX-354-5), basically developed (as the TX-354-3) in 1964 for the Scout second stage, among other applications, Thiokol kept the steel case used on Castor I but substituted carboxy-terminated polybutadiene for the polybutadiene-acrylic acid used as the binder for the earlier version, keeping aluminum and ammonium perchlorate as fuel and oxidizer. This increased the specific impulse from under 225 for the Castor I to more than 235 lbf-sec/lbm for Castor II and the total impulse from 1.63 million to 1.95 million pounds, improving the payload capacity for the Thorad by 20 percent over the TAT.40
A further major advance in propulsion technology for the Scout came in 1977-79 when, under contract to the Vought Corporation, Thiokol produced a new third-stage motor at its Elkton Division in Maryland. This was the Antares IIIA (TE-M-762, Star 31), employing a HTPB-based binder combined with ammonium perchlorate and aluminum. This propellant increased the specific impulse from about 285 for the composite-modified double-base propellant used by Hercules in the Antares IIB to more than 295 lbf-sec/lbm. In addition to the higher-performance propellant, Thiokol used a composite case made of Kevlar 49 and epoxy. Introduced commercially in 1972, Kevlar 49 was DuPont’s registered trademark for an aramid (essentially nylon) fiber that combined light weight, high strength, and toughness. Lighter than fiberglass, it yielded a mass fraction of
FIG. 7.6
Scout S-131R on August 10, 1965, with a new Castor II second stage. (Photo courtesy of NASA)
|
|
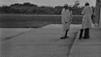
® 1965-L-06I38
|
|
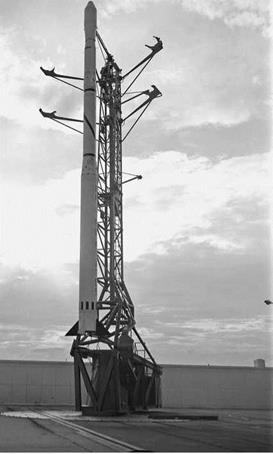
0.923 compared with Antares IIB’s already high 0.916. Burning much longer than the propellant in the Antares IIB, the one in the Antares IIIA produced a lower average thrust, but its total impulse at the high altitudes in which it operated was 840,000 pounds compared with 731,000 pounds for the Antares IIB. No doubt because of the higher erosive propensities of the Antares IIIA motor, which had a higher chamber pressure than the Antares IIB, the newer motor used 4-D carbon-carbon (pyrolitic graphite) for the nozzle-throat insert.41
Launched initially at the end of October 1979, the Scout version G1 with the Antares IIIA as its third stage appears to have been the first launch vehicle to use an HTPB propellant, but the first use of the substance may have been on an improved Maverick tactical (air-
to-surface) missile. Thiokol provided the Maverick’s initial motor, with development starting in 1968, but under contract to the Air Force Rocket Propulsion Laboratory at Edwards AFB, Aerojet had begun in August 1975 to develop the improved motor with HTPB propellant. By October 1976, Aerojet had produced 12 demonstration verification motors. Aerojet did get production orders for some version of an improved Maverick motor. But interestingly, an organization called the ATK Tactical Systems Company, producer of the Maverick heavy warheads, later claimed to be under contract to provide a rocket motor very similar to the Aerojet design with an HTPB propellant, an HTPB liner, an aluminum case, an 11-inch diameter, and a glass phenolic exit cone, all features of Aerojet’s motor.42
Another motor that used HTPB was Thiokol’s Star 48, used on the Payload Assist Module (PAM)—a third stage on the Delta and an upper-stage motor used from the Space Shuttle. Thiokol began developing the motor in 1976. The firm made the Star 48 motor case with titanium and used the recently developed advanced composite, carbon-carbon, for the nozzle’s exit cone. The PAM was an offshoot of Minuteman, stage three, which Thiokol began producing in 1970 essentially using the original Aerojet design. The purpose of the PAM on the shuttle was to propel satellites from a low parking orbit (about 160 miles above Earth) to a higher final orbit, including a geosynchronous transfer orbit. It used the same basic HTPB-aluminum-ammonium perchlorate propellant as Thiokol’s Antares IIIA rocket motor.43
The Inertial Upper Stage (IUS) featured a much more problematic set of motors using HTPB propellant. Designed under management of the Space and Missile Systems Organization (SAMSO—a recombination of the air force’s Ballistic and Space Systems Divisions) primarily for use with the Space Shuttle (for when orbiter payloads needed to be placed in geosynchronous orbit), IUS became a difficult stage to develop for a variety of complicated reasons. Many of them were technical, but the major ones involved management. Some, but far from all, of the management problems resulted from the fact that the IUS, which initially stood for Interim Upper Stage, was conceived as a temporary expedient until a more capable Space Tug could fly with the shuttle. When the Space Tug was not terminated but “just slid out year-by-year under budget pressure," as one air force general expressed it, the IUS shifted from being a minimal modification of an existing upper stage such as Transtage or Agena to become, starting about 1978, a projected “first line vehicle in the Space Transportation System." Yet “considerable cost reduction pressure [remained ] as an outgrowth of the interim stage thinking."
Moreover, the air force was developing the vehicle under “a contract structure which strongly incentivized performance, but only provided limited cost incentives."44
The IUS ultimately overcame its birth pangs to become “an integral part of America’s access to space for both military and civilian sectors." It had its beginnings in 1969 when presidential direction gave impetus to studies leading to the Space Shuttle. Since the shuttle would be incapable of reaching geosynchronous and other high 282 orbits, the IUS ultimately became the solution. The DoD agreed to Chapter 7 develop it, proposing in 1975 that it use solid propellants to hold down costs.
In August 1976, the air force selected Boeing Aerospace Company as the prime IUS contractor. The contract provided incentives for meeting performance and cost targets, but Boeing was liable for only 10 percent of cost overruns. Moreover, cost projections for IUS had been based on assumptions, according to Maj. Gen. William Yost of the air force, that “the M-X and Trident missile programs would develop most of the solid rocket motor technology. . . needed by the IUS. Unfortunately, the schedules for those programs slipped far enough that the IUS program became the leader in developing the solid rocket motor technology necessary to meet our performance requirements." This led the contractor to increase “spending to insure that he will achieve his performance goals and earn the performance fees." As a condition of revising the contract with Boeing in 1979, the air force insisted that the firm’s apparent “management deficiencies be resolved," and Boeing appointed a new program manager, assigned senior managers to oversee major subcontractors, and instituted formal review to correct the problems.45
Boeing had begun a planned 18-month preliminary design phase in August 1976 when it won the contract, to be followed by a 28- month development phase. This would have made the IUS available by June 1980. Soon after winning the basic contract, Boeing subcontracted with CSD to design and test the solid motors to be used in the IUS. CSD chose to use a hydroxy-terminated polybutadiene propellant, as had Thiokol in the Antares IIIA motor for Scout. CSD selected a carbon-carbon material for the nozzle, which would be manufactured using a new process that held costs to a low level. It was making the case out of Kevlar. Thiokol was also using the same or similar materials on the contemporary Antares motor, raising questions about the extent to which CSD was taking the lead “in developing the solid rocket motor technology" needed for the IUS (as Yost claimed), but it appears that CSD and Thiokol
were, in effect, competing for that lead from 1977 to 1979, with Thiokol winning the contest.46
At first things seemed to be going well with motor development. CSD conducted a series of tests in 1977 to prove the adequacy of the nozzle and motor. It subjected the nozzles to successful 85-second tests at the Air Force Rocket Propulsion Laboratory on June 10 and July 15. A follow-on 145-second test of the nozzle at the laboratory on October 7 was again successful. Moving to the Arnold Engineering Development Center (AEDC), CSD subjected a full-scale motor with 21,000 pounds of propellant to a 154-second test, which it completed successfully. On May 26, 1978, a further test of the nozzle material using the carbon-carbon made with the new, costsaving technique again occurred without problems in a 140-second test firing at the Rocket Propulsion Laboratory.
But on October 19, 1978, a test of the Kevlar case at AEDC resulted in its bursting at only 750 pounds per square inch of water pressure instead of CSD’s prediction of 1,050 pounds. The firm decided that defective manufacturing equipment caused the failure. After redesigning the equipment and strengthening the structure of the case, AEDC conducted six more tests between January and September 1979. Five of them were successful, with the cases withstanding higher pressures than specified. By this time, the design of the IUS had evolved into two stages with similar larger and smaller motors. A test firing of the large motor was scheduled on October 17, 1978. Inspection of the motor revealed some propellant that was improperly cured, resulting in softness and blistering, delaying the test. With the propellant recast, the test occurred on March 16, 1979, with a 145-second firing that generated more than 50,000 pounds of thrust. Engineers vectored the nozzle several times, demonstrating its ability to direct the thrust for course corrections. A follow-on test of the small motor occurred on June 25, again with the nozzle moving. Both tests were successful.47
Most other tests in 1979 went well in most respects, but cracks had appeared in the nozzle of the small motor. Moreover, a special feature of the nozzle for the smaller motor was an extendable exit cone, which was added to the design in 1978. This was a series of conical pieces that in the final design (as of 1983) telescoped out (extended) and fit over one another to increase the nozzle expansion ratio from 49.3:1 without the extension to 181.1:1 with the pieces extended. Although the design, which would be used only on some missions, increased the weight of the motor, it added about 15 lbf- sec/lbm to the specific impulse. Unfortunately, about half the exit
cones for the small motors were defective. Finally, five motors proved to have more “bad" propellant. Boeing and CSD said they could still be tested, but Aerospace Corporation, advising SAMSO, disagreed.
On October 1, 1979, SAMSO formed a tiger team of experts from several organizations (including NASA, the Rocket Propulsion Laboratory, and Aerospace Corporation) to investigate technical concerns and management. This resulted in the management changes at Boeing already mentioned and a change in one supplier. CSD had 284 been making the large Kevlar motor cases, and Brunswick Corpora – Chapter 7 tion made the small one, which the team found to be superior. As a result, Brunswick became the supplier of both sets of cases.
During 1980, the production team solved the other problems. For example, the cracks in the nozzle of the smaller motor proved to result from unequal expansion of two materials. A silica-phenolic insulation material expanded faster than the carbon-carbon next to it. The solution was to wrap the silica phenolic with graphite to limit expansion. The problem with the exit cones resulted from the methods of the supplier, Kaiser, still learning about the properties of carbon-carbon. A change in tooling and ply patterns plus improved quality-control procedures provided the solution. The degraded propellant had all come from a single batch and was usable in tests. As a result, three rocket motor tests of each motor (small and large) during 1980 at the AEDC were successful. (All of these development tests at AEDC simulated conditions the motors would actually face in flight at altitude.) There were further problems with propellant cracks, delamination of the carbon material in the extendable exit cones, and the mechanism for extending the exit cones, but engineers solved them, too.48
The various technical and managerial problems had led to more than two years of delay and to cost overruns that basically doubled the originally projected cost of the IUS. Although many of the problems resulted from contractual arrangements and the initial, interim character of the upper stage, many of them involved fabrication methods and quality control. They showed that despite more than two and a half decades of continuous rocket development, rocket engineering in the United States still required constant attention to small details and, where new technology was involved, a certain amount of trial and error, although Thiokol’s success with Antares IIIA showed that sometimes the process of innovation could go more smoothly. (But not always, as Thiokol’s later problems with the shuttle solid-rocket boosters showed.) Because the IUS was designed principally for use on the Space Shuttle, NASA’s
delays with that program made the stretch-out of the IUS schedule less problematic than it could have been.49
On October 30, 1982, the first Titan 34D and the first IUS together successfully launched a Defense Satellite Communications Satellite II and the first DSCS III into geosynchronous orbit from Cape Canaveral. As planned, the second-stage burn achieved low – Earth orbit, with the first IUS motor carrying the third stage and the satellites into transfer orbit. The second IUS motor placed the payloads in geostationary orbit, with hydrazine thrusters making final adjustments in the placement of each satellite. During launch the telemetry failed, attributed to a leak in the seal of a switch. But the guidance/control system, flying “blind" (without telemetry) or external control from Earth autonomously carried out the provisions of the flight plan, as designed.50
As completely designed, the IUS was roughly 17 feet long and had a maximum diameter of 9.25 feet. Fully loaded, the large, first – stage motor (SRM-1) carried 21,400 pounds of ammonium perchlo – rate-HTPB-aluminum propellant, but the propellant load could be reduced as required for specific missions (as was done with the first launch). The smaller, second-stage motor (SRM-2) could carry up to 6,000 pounds of the same propellant. The propellant-delivered specific impulse of SRM-1 was upward of 295, that for SRM-2 about 290, increased to more than 300 lbf-sec/lbm with the extendable exit cone.51
To mention just one other use of an HTPB propellant, this technology came to the Delta with the Castor IVA strap-ons. A Castor IV (TX-526) had actually replaced the Castor II strap-ons in December 1975 for the Delta model 3914, but it was a reversion from the carboxy-terminated polybutadiene used in the older strap-on to polybutadiene-acrylic acid (PBAA) as the binder. The reason for the shift may have been cost, since the Castor IV at 29.8 feet long and 40 inches in diameter contained much more propellant than the 19.8-foot by 31-inch Castor II, and CTPB was more expensive than PBAA. But in the early 1980s, Goddard Space Flight Center (manager of the Delta program) shifted to an uprating with the Castor IVA. Tested and qualified in 1983, the new motors were not introduced then because of the impending phaseout of the Delta in favor of the Space Shuttle. With the post-Challenger resurrection of expendable launch vehicles, McDonnell Douglas proposed incorporating the Castor IVAs on Delta II as a low-risk improvement. The new strap-ons kept the steel case and graphite nozzle throat material. But they used the HTPB-aluminum binder with a higher loading of
FIG. 7.7
The Inertial Upper Stage attached to the Magellan spacecraft in the payload bay of Space Shuttle
Atlantis. (Photo courtesy of NASA)
|
|
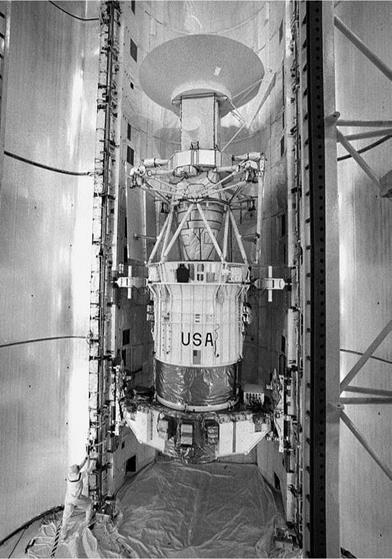
solids. This increased the average thrust for the same-sized motor from 85,105 to 98,187 pounds.52
Analysis and Conclusions
Although the solid-propellant breakthrough achieved by the Polaris and Minuteman programs provided many technologies to launch vehicles, others followed. These included the carbon-phenolic throat, segmenting, and the tang-and-clevis joints for the Titan SRM; Flex-
seal nozzles used on the Space Shuttle’s huge solid-rocket boosters; and the use of HMX in the propellant for the Antares IIA stage of the Scout launch vehicle. Although it apparently found its first use on an improved motor for the Maverick tactical missile, HTPB propellant seems to have first appeared on a launch vehicle in the Scout G1.
Although sometimes innovations occurred without many apparent problems, as in Thiokol’s use of HTPB in the Scout’s Antares IIIA, the IUS, employing many similar technologies, faced a whole host of difficulties, many of them technical. The field joints for the shuttle caused the Challenger tragedy, and when Hercules developed the solid-rocket motor upgrade for the Titan IVB, technical problems delayed launch of the first uprated launch vehicle until well beyond the period covered by this history. Rocket engineers continued to advance the state of their art, but often they could do so only by trial and error. There was no such thing as a mature rocket science that could guide them effortlessly through the design of new technologies, but accumulated experience, data, computers, instrumentation, and telemetry allowed practitioners to solve most problems.
DURING THE LAST 45 YEARS, LAUNCH VEHIcles have propelled countless spacecraft and satellites into space, in the process revolutionizing life on planet Earth. Americans have become dependent upon satellites for everything from what they watch on television to how they wage war. Space telescopes and other spacecraft have greatly expanded our knowledge of the universe. What enabled the United States to develop the technology for access to space so quickly? One major contributor was the cold war, whose terminus is the end point for this book. Had it not been for the Soviet threat, symbolized by Sputnik, the enormous expenditures needed to develop U. S. missiles, launch vehicles, and satellites would have been lacking.1
There seems to be no accurate compilation of total expenditures on missiles and launch vehicles during the cold war. Obviously, however, the outlays were enormous and constituted a virtual sine qua non for the speedy development of the technology. An early (1965) estimate of the total costs for ballistic missiles to that point in time suggested a figure of $17 billion, equal to some $106 billion in 2005 dollars. Including missile sites, which were irrelevant to launch vehicles, this figure also covered factories for producing propellants, engines, airframes, and guidance/control systems; test facilities; ranges with their testing, tracking, and control equipment; laboratories; and much else.2 A further indicator of the huge costs of missiles and launch vehicles was the $9.3 billion (nearly $55 billion in 2005 dollars) spent on the Saturn launch – vehicle family.3
Fears of Soviet missile attacks and the spending they stimulated were one factor in the development of launch-vehicle technology. They also provided the context for a second major contributor, the work of heterogeneous engineers in stimulating Congress, several presidential administrations, and the American people to invest the money needed

Monkey Baker posing with a model of a Jupiter vehicle, one of which launched it into space in an early example of the use of a missile as a launch vehicle, part of the space race inaugurated by the Soviets’ launch of Sputnik. (Photo courtesy of NASA)
for rapid development. Without individuals like Trevor Gardner, John von Neumann, Bernard Schriever, Theodore von Karman, Wernher von Braun, and William F. “Red" Raborn, funding for missiles and rockets (with their frequent failures in the early years) would not have been forthcoming.
As missiles and launch vehicles increased in size and complexity, it is not surprising that many of them experienced failure. Americans recognized the arcane nature of the technology by their use of the term “rocket science" to describe it. Ironically, the rocket “scientists" could not always predict the problems the technology encountered in operation. Methods of testing rockets and missiles, technical reports, computer tools, and other supporting infrastructure continued to grow. But as recently as 2003, when the Columbia disaster occurred, NASA discovered once again that it did not fully understand all aspects of rocket behavior despite extensive experience. Hardly an isolated case, this major accident simply reemphasized that predictability of rocket behavior had been problematic from Robert Goddard’s inability to reach the altitudes he had forecast until very near the present. If we define rocket science as a body of knowledge complete and mature enough to allow accurate predictions of problems, then clearly, such a science does not exist. Maybe it will someday, but what we currently have is rocket engineering.
Of course, there are other ways of defining science. And recent science has hardly been immune from uncertainties, such as those
regarding the big bang theory about how the universe arose. Moreover, the success of rocket developers in resolving unanticipated problems and getting their creations to work certainly compares 290 favorably with scientists’ accommodations of unexpected data by Chapter 8 adjustment of theories. The difference lies in science’s basic quest to understand the universe as compared with rocket engineers’ effort to make their vehicles meet design goals. These engineers used any available resources to reach that end, including science. Certainly the engineers, especially those engaged in developing engineering theory (often called engineering science), wanted to understand how rockets worked. But often they had to “fix" problems in the absence of such fundamental understanding. In such cases, they had to resort to trial and error, finding a solution that worked without necessarily understanding why it worked. Accumulated knowledge, engineering theory, and intuition helped in correcting problems, but the solution did not always work when a particular technology had to be scaled up to a larger size. Sometimes, in fact, innovative solutions ran counter to existing theory.
This reality shows that the basic process of developing rockets constituted engineering, not science. Such an argument tallies with the general theses of Edwin Layton, Walter Vincenti, and Eugene S. Ferguson about engineering in general as different from science— especially their points about engineers’ focus on doing as opposed to scientists’ knowing, on the importance of design for engineers, and on the role of art in that design. Vincenti, especially, proposed more historical analysis of the ways engineers sometimes must make decisions in the absence of complete or certain knowledge.4
Research for this book did not start with the thought of applying Vincenti, Layton, and Ferguson’s arguments to rocket technology. Instead, as I gathered information about the process of missile and launch-vehicle development, I became increasingly convinced that it fit the mold of engineering, not science. This is particularly true in the areas of injection, ignition, and propulsion of liquid propellants and of combustion instability in solids. Problems in these areas occurred in the design of the V-2, the H-1, F-1, and space shuttle main engines as well as many solid-propellant motors. A. O. Tischler, NASA assistant director for propulsion, in 1962 called injector design “more a black art than a science."5 With the passage of time, the art became less “black," but art it remained.
Problems with rocket design were not exclusive to propulsion. As early designs had to be scaled up or modified with new and better materials to improve performance, unanticipated problems continued to occur through the end of the period of this book and be-
yond. Failure to understand the behavior of foam covering the Space Shuttle’s external tank as it rose through the atmosphere continued beyond the Columbia disaster. Problems in developing the solid – rocket motor upgrade for the Titan IVB persisted beyond the end of the cold war. These and other instances demonstrate the continuing uncertainties accompanying rocket engineering, especially in an environment where speed and cost control limited basic research.
In the face of this unpredictability, it is noteworthy that missile and launch-vehicle technology evolved as quickly as it did. Design and development engineers did exceptionally well to find innovative solutions to problems and allow the technology to advance as successfully as it did.
Another key to the speedy development of rocket technology was the process of innovation. Sadly, known sources often shine little light on the individuals or processes involved. Interviews and correspondence with rocket engineers sometimes yield information.6 But even the principals in a particular development frequently cannot remember who came up with a discovery or how it came about. Engineers typically worked in large teams to design rocket systems or components. And many innovations involved more than one firm. Otto Glasser at the Western Development Division offered an interesting analogy for the difficulty of finding out who contributed significantly to innovation under such circumstances: “If you were to back into a buzz saw could you tell me which tooth it is that cut you?"7
Many innovations did not arise from initial design but occurred in response to problems during testing. Examples of these that seem to fit Glasser’s “which tooth?" analogy include the process of rollring forging developed by UTC, Westinghouse, and the Ladish Company and UTC’s tape-wrapped, carbon-phenolic nozzle throat for the Titan solid-rocket motors. The companies doing the innovating are clear, but we do not know which individuals were the principal innovators. How Aerojet engineers fixed problems with the Trans – tage (ranging from a weakness in a nozzle extension to malfunctioning bipropellant valves) likewise remains somewhat mysterious. Regardless, all of these technologies appear to exemplify trial-and – error engineering.
What we do know about innovations in rocket development suggests that they did not follow a single pattern. Hugh Aitken provided a felicitous description in his book about radio technology, saying that it involved “a process extending over time in which information from several sources came to be combined in new ways."8 In the case of missiles and launch vehicles, large numbers of firms, institutions, and organizations helped provide the requi-
site information. Among those that contributed were firms such as Aerojet, Rocketdyne, Pratt & Whitney, Douglas, the Martin Company, UTC/CSD, and Thiokol; and other organizations like the air 292 force’s Western Development Division and its successors, the ar – Chapter 8 my’s counterparts at Redstone Arsenal, the navy’s special projects office and its successors, NASA Headquarters and various centers (notably JPL, Langley, Lewis, and Marshall for rocketry), the Naval Ordnance Test Station and its successors, the Air Force Rocket Propulsion Laboratory (under various names), the Arnold Engineering Development Center, the Chemical Propulsion Information Agency (CPIA) and its predecessors, and the Armour Institute (later Illinois Institute of Technology).
During developmental planning, representatives from entities like these met to exchange ideas and information. Then, if problems arose for which there were no known explanations and/or no evident solutions, as often happened, engineers and other experts from perhaps different organizations met to brainstorm and troubleshoot. With the enduring problem of combustion instability, for instance, numerous university researchers, as well as other engineers, have long been seeking both understanding and solutions.9 Surviving sources indicate that the general process was often complex, with no record of specific contributors except the authors of technical reports. But the authors themselves often wrote in the passive voice, masking individual participants beyond the authors themselves, who presumably were involved. Engineers sometimes remembered (but how accurately?) some details of solutions but not always the precise process.
Among the factors that conditioned rapid development of missile technology, the existing literature points to interservice competition, often as a problem but also as a spur to innovation.10 Virtually unnoticed in the literature but probably more important was interservice and interagency cooperation. The CPIA was a key promoter of cooperative exchange of information, but not the only one. For instance, the air force saw the navy’s Polaris program as a competitor for roles, missions, and funding. The Polaris competition encouraged the air force’s development of its own solid-propellant missile, Minuteman. Yet ironically, Polaris itself might not have been possible without technologies the air force developed. Min – uteman, in turn, borrowed the use of aluminum as a fuel from the navy. Likewise, the air force reluctantly accepted NASA as a developer of rocket technology, and the army was not happy to lose the von Braun team and JPL to the civilian space agency. Both services, however, cooperated with NASA (and vice versa), with the air force
FIG. 8.2
A static test of a Space Shuttle solid-rocket booster at the Morton Thiokol test site in Wasatch, Utah, on January 20, 1989. (Photo courtesy of NASA)
|
|
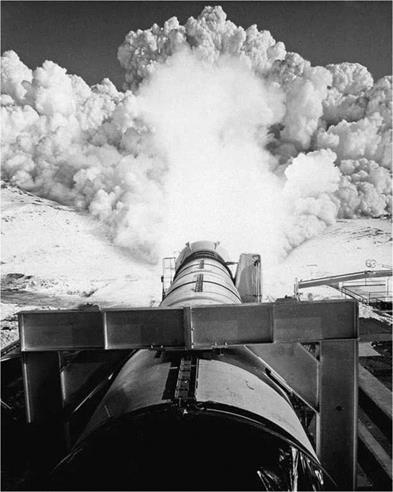
loaning important managers like Samuel Phillips to help NASA with its programs. Moreover, many astronauts came to NASA from the U. S. Marine Corps, the navy, and the air force.11
Technology transfer also contributed to rapid rocket development, but its details remained almost as elusive as those involving innovation. Federal contracting agencies often precluded contractors from treating innovations developed under government contract as trade secrets or company property. Lockheed, for example, was unable to protect its Lockseal technology, permitting Thiokol to increase its size and use it to vector the exhaust from the shuttle solid-rocket boosters under the name Flexseal.
Engineers frequently learned about the rocket technology of one firm or organization, established their credentials, and moved to an-
other organization, carrying their knowledge with them. This helped transfer technology and promote overall rocket development. For instance, after Charles Bartley developed early rubberized, compos – 294 ite solid propellants at JPL, he founded the Grand Central Rocket Chapter 8 Company, which participated in solid-rocket development and then became part of Lockheed Propulsion Company in 1960-61.12 Bartley also transferred JPL technologies to Thiokol when it entered the rocket business. Barnet R. Adelman had worked with both liquid and solid propellants at JPL. He then became technical director of the Rocket Fuel Division at Phillips Petroleum and then director of vehicle engineering for the Ramo-Wooldridge Corporation. At Ramo-Wooldridge, he became a major supporter of the Minuteman missile, along with Col. Edward N. Hall. Next, he helped found United Research Corporation (later UTC/CSD). The knowledge he carried with him undoubtedly helped UTC develop the solid-rocket motors for the Titan III and IVA.13 Adelman and other UTC executives’ knowledge of people in the solid-propellant field also enabled them to hire other experienced engineers who furthered the success of the firm.
The previous chapters have covered many other examples of technology transfer through people moving from one organization to another. A well-known example was the von Braun team, including Krafft Ehricke, who brought German V-2 technology to the United States. Importantly, though, the technology they transferred was only part of the story. Americans, notably Rocketdyne engineers, learned much from the V-2 and its German developers, but they went on to create major innovations of their own in developing successor engines, extending from the Redstone to the space shuttle main engine. This synergism contributed in complex ways to American rocket development.
Management systems constituted another factor in the rapid development of missile and launch-vehicle technology. Without such systems (and the systems engineering they fostered), the various components of rockets would not have worked together with increasing reliability to launch their payloads as time passed. Such systems (and the individual management skills that complemented and implemented them) were especially necessary as the numbers of people from industry, government, and universities increased and became interdependent. Growth was rapid. The Atlas missile had only 56 major contractors in 1955. By decade’s end, the number had escalated to roughly 2,000. To keep this many organizations on schedule and to ensure quality control, Gen. Bernard Schriever availed himself of a systems engineering-technical direction con-
tractor (Ramo-Wooldridge). Beyond that problematic arrangement, the Western Development Division instituted a management-control system to keep track of schedules and to deal with problems as they arose. Graphs, charts, and computer tracking permitted Schriever and his successors to keep their projects more or less on schedule.
The enormously complex Polaris system, likewise, led Adm. “Red" Raborn of the navy to oversee development of the Program Evaluation and Review Technique, analogous to Schriever’s system. Critics complained about both systems, but without some such arrangements, early missile development could hardly have been as successful and rapid as it was.
George Mueller and Sam Phillips brought these kinds of systems to NASA, enabling it to land astronauts on the Moon in less than a decade from President Kennedy’s 1961 exhortation to do so. These systems allowed Apollo to stay on schedule and within budget while still achieving configuration control. The overall success of missile and launch-vehicle development owed much to such arrangements.
The basic processes of rocket engineering did not change abruptly with the end of the cold war about 1991. But the context in which research and development had to occur suffered a drastic shift. It became less urgent for new technologies to appear, while Congress exercised stringent oversight over costs and schedules. Basing itself on studies in the late 1980s and early 1990s, the air force responded to the new environment with the Evolved Expendable Launch Vehicle (EELV) program. This replaced Titan II, Delta II, Atlas II, and Titan IV with a new series of boosters capable of launching 2,500 to 45,000 pounds into low-Earth orbit with a 98 percent reliability rate. This exceeded capabilities of previous launch vehicles at costs 25 to 50 percent below earlier figures.
The air force provided four $30 million contracts in August 1995 to Lockheed Martin, Alliant Techsystems (which acquired Hercules Aerospace Company, the former Hercules Powder Company, in March 1995), Boeing, and McDonnell Douglas to develop proposals for the EELV vehicles. Out of this process, McDonnell Douglas (later acquired by Boeing) and Lockheed Martin won contracts for actual development.14 The further development of this program is beyond the scope of this book, but the Lockheed Martin Atlas V’s successful launch on August 21, 2002, and that of Boeing’s Delta IV on November 20, 2002, suggested that the EELV launchers would capture most of the military market. The use of a Russian RD-180 engine on the Atlas V symbolized the radical change that had occurred
since the end of the cold war. The air force reportedly believed as of August 2002 “that through 2020 the two new EELV families w[ould] reduce the cost per pound to orbit to $7,000 compared with $20,000 296 for the old booster fleet," saving “$10 billion in launch costs"—a Chapter 8 50 percent reduction “compared with launching the same military
payloads on old Delta, Atlas and Titan boosters."15
Similar concerns about cost affected NASA’s efforts to develop new launch vehicles.16 NASA also had to contend with concerns about safety after the Columbia disaster. In 2005-2006, the agency developed a concept for a safer pair of launch vehicles that would use technologies from the Space Shuttle or other existing hardware without the problems of an orbiter with wings that could be struck by debris from an external tank. A crew launch vehicle (named Ares I) would consist of one enlarged solid-rocket booster derived from the shuttle, a stage powered by a Rocketdyne J-2X engine (evolved from the J-2 used on the Saturn V), and a crew capsule at the top of the stack, equipped with an escape rocket to separate the crew from the launch vehicle in the event of problems. In this Apollo-like capsule, the crew would face little danger from debris separating from the shuttle on launch, as happened with tragic consequences during the Columbia launch in 2003 and again (without significant damage) during the launch of Space Shuttle Discovery in 2005 and subsequent shuttle launches in 2006.
For future space exploration, NASA also planned a heavy-lift launch vehicle named Ares V with two solid-rocket boosters, a central element derived from the shuttle’s external tank, five RS-68 engines modified from the Delta IV, and an earth-departure stage propelled by a J-2X engine. Like Ares I, this vehicle would be configured in stages, but the first-stage solid-rocket boosters would flank the central booster tank, with the liquid engines above the tank. Ares V thus resembled a Titan IV in its general configuration. NASA calculated that these two future vehicles would be 10 times as safe as the shuttle and would not cost as much as a completely new vehicle because of the use of proven technologies.17 However, both vehicles were in a state of development and could easily change as further possibilities came under study.
It would be foolhardy to predict how the struggle for cheaper access to space will play out in the new environment ushered in by the fall of the Soviet Union. However, maybe this history of the uncertainties and difficulties of developing launch vehicles in a different environment will highlight the kinds of problems rocket engineers can expect to encounter in a more cost-constrained atmosphere. Rocket reliability improved significantly in the 50 years or
so following the serious beginnings of U. S. missile and rocket development. Recently, launch vehicles have experienced only a 2-5 percent failure rate. By comparison, the first 227 Atlas launches failed 30 percent of the time. Nevertheless, in 2003 the Columbia Accident Investigation Board stated, “Building and launching rockets is still a very dangerous business and will continue to be so for the foreseeable future while we gain experience at it. It is unlikely that launching a space vehicle will ever be as routine an undertaking as commercial air travel."18 Knowledge of this reality, coupled with the historical experiences recounted previously, may help contemporary rocket engineers design both cheaper and better launch vehicles. Perhaps Congress and the American people can also benefit from knowing the kinds of challenges rocket engineers are likely to face.