Apollo 12
Date: 14-24 November 1969
Crew: Commander – Pete Conrad
CM Pilot – Dick Gordon LM Pilot – Alan Bean
Command Module: Yankee Clipper Lunar Module: Intrepid
Accomplishments: Pinpoint landing within 535 feet of Surveyor 3, two extended Moonwalks, setup and initiated Geophysical Station and Lunar Nuclear Power Station.
J. L. Chen, How to Find the Apollo Landing Sites,
The Patrick Moore Practical Astronomy Series, DOI 10.1007/978-3-319-06456-7_4, © Springer International Publishing Switzerland 2014
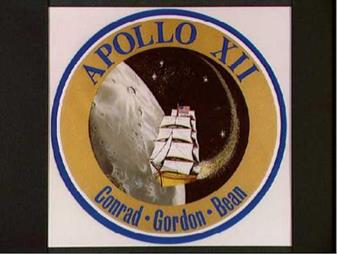
Fig. 4.1 Apollo 12 Insignia. Courtesy of NASA
|
Early in Apollo program planning, NASA defined a sequence of missions:
• “A” missions were unmanned tests of the launch vehicles and the Command Module
• “B” missions were unmanned tests of the LM
• “C” missions (Apollo 7) were manned, Earth orbital tests of the Command Module
• “D” missions (Apollo 9) were LM/CSM tests in Earth orbit
• “E” missions (none were flown) were tests in high Earth orbit
• “F” missions (Apollo 10) were lunar orbit tests
• The “G” mission was the Apollo 11 landing.
• “H” missions (Apollo 12, 13, and 14) would be subsequent flights to other landing sites using the basic equipment
• “I” missions (none flown) would be lunar orbit-only science flights
• “J” missions would be the longer visits that LM design changes would make possible. Equipped with Lunar Roving Vehicles (LRV), the J-mission crews would be able to extend their range on the lunar surface and visit a variety of geologic features some distance away from the landing site, collect greater quantities of rock and soil samples, utilize a greater number of tools carried on the Rover, and LRV would serve as a mobile experiment platform. In general, the J-missions promised a significant increase in productivity. However, before the J-missions could be attempted, NASA had to demonstrate that crews could achieve pinpoint landings, work a full-day in the stiff suits and, if necessary, walk several kilometers back to the LM from a disabled Rover.
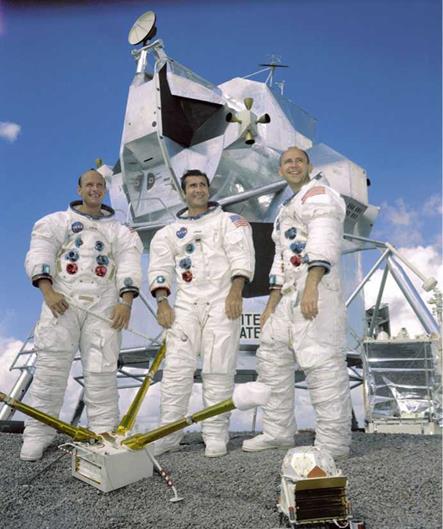
Fig. 4.2 The Apollo 12 team. Courtesy of NASA
|
Apollo 12 represented the first of the H missions. The Apollo 12 mission was presented with a couple of challenges not faced by Apollo 11, one known prior to launch and one occurring during launch.
The particular challenge for the Apollo 12 mission was the achievement of a pinpoint landing. One of the significant discoveries prior to the Apollo landings was the existence of mass concentrations, or mascons, on the Moon. The lunar mascons were discovered by scientists at the NASA Jet Propulsion Laboratory (JPL) in 1968 from analyzing the orbital and navigational data from the NASA’s Lunar Orbiter spacecraft. The analysis established a correlation between very large positive gravity anomalies and depressed circular basins on the moon. Many of the Apollo experiments on the lunar surface tried to collect data to explain the gravity variations.
NASA had to establish a special team of scientists and engineers to explain why the Lunar Orbiter spacecraft being used to test the accuracy of the Apollo navigation system were experiencing errors. The mission specification required an accuracy of 200 meters, whereas the test results predicted a navigational position of 2,000 meters, ten times the allowed specification error. This meant that the predicted landing areas were 100 times as large for reasons of safety, and posed a challenge to precise lunar landings. The principle cause for these lunar orbital errors was due to the strong gravitational perturbations of the mascons. NASA was able to compensate for the existence of the mascons in the Apollo navigation software, and this compensation was first applied to Apollo 12. The Apollo 12 landing was within 163 meters of the target, the Surveyor 3 spacecraft.
During the launch of Apollo 12, the spacecraft and Saturn V were twice struck by lightning, causing a momentary power crisis aboard Yankee Clipper that almost aborted the mission. Apollo 12 launched on schedule from Kennedy Space Center, during a rainstorm. 36.5 seconds after lift-off, friction from the vehicle passing through the rainstorm triggered lightning through the launch vehicle, through the Saturn’s ionized plume, and down to the ground. The protective circuits of the fuel cells in the Service Module (SM) took all three fuel cells offline because the lightning caused a surge that falsely indicated overloads. The cascading effect of the lightning strike also disabled much of the Command/Service Module (CSM) instrumentation. A second strike at 52 seconds after launch knocked out the attitude control. The telemetry stream at Mission Control was totally disrupted. However, the vehicle continued to fly correctly; the strikes had not affected the Saturn V Instrument Unit.
The loss of all three fuel cells put the CSM entirely on batteries. Apollo 12 power systems were unable to maintain normal 28 V DC bus voltages into the heavy 75 amp launch loads. One of the AC inverters dropped offline. As a result, these power supply under volt conditions lit nearly every warning light on the control panel and caused much of the instrumentation to malfunction.
Legendary NASA “steely-eyed missile man” John Aaron was the EECOM at Mission Control for the launch. Aaron remembered a similar telemetry failure pattern from an earlier test when a power supply malfunctioned in the CSM Signal Conditioning Equipment (SCE). The SCE converts raw signals from instrumentation to standard voltages for the spacecraft instrument displays and telemetry encoders.
Aaron made a call to the Apollo 12 crew, “Try SCE to aux.” This switched the SCE to a backup power supply. The switch was fairly obscure and neither the Launch Flight Director Gerry Griffin, CAPCOM Gerry Carr, nor Commander Conrad immediately recognized it. Lunar Module Pilot ( and future Apollo artist) Alan Bean, flying in the right seat as the CSM systems engineer, remembered the SCE switch from a training incident a year earlier when the same failure had been simulated. Aaron’s and Bean’s quick recall saved the mission and prevented an abort. Bean put the fuel cells back on line, and with telemetry restored, the launch continued successfully. Once in earth orbit, the crew and Mission Control reviewed all systems and declared all systems go prior to trans-lunar injection. The lightning strikes had caused no serious permanent damage and the crew, with the onboard power systems restored, were able to continue the mission.
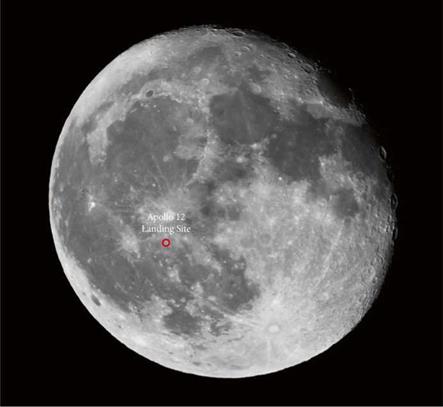
Fig. 4.3 Apollo 12 and the Ocean of Storms. Courtesy of the author
|
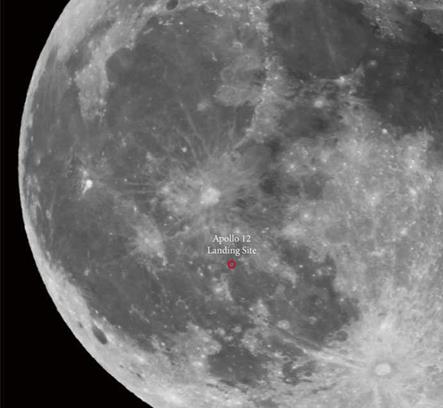
Fig. 4.4 First Zoom-in of Apollo 12 landing site. Courtesy of the author
|
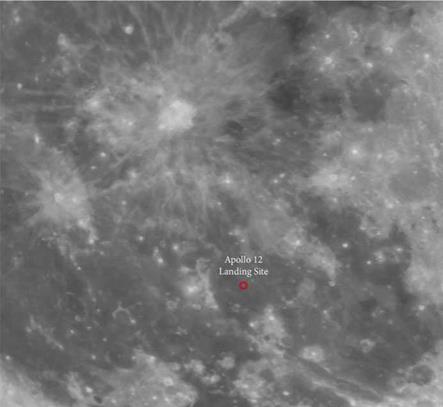
Fig. 4.5 Second Zoom-in of Apollo 12 and Surveyor 3 site. Courtesy of the author
|
The key to sighting the Apollo 12 landing site is first recognizing the great Copernicus crater. Copernicus is one of the most easily identifiable features on the Moon, located south of Mare Imbrium, or the Sea of Rains. Through a small telescope, one can make out the layered nature of Copernicus, and outward “rays” of light and dark material splashed by the impact of an object that created the crater eons ago. South of Copernicus and past a smaller crater known as Reinhold, is the small Ocean of Storms and the site of Apollo 12. NASA’s conservative approach to landing site selection resulted in another rather featureless landing zone that can be appreciated, but the actual site is difficult to pinpoint for the backyard observer. Apollo 12 landed near the Surveyor 3, near the edge of a small crater. However, the Surveyor 3 and the crater are beyond the resolution of most backyard telescopes.
As with Apollo 11, conservative safety considerations dominated the criteria for landing site selection for Apollo 12. The Ocean of Storms site was chosen because it had few craters and boulders, with less than 2° slope in the approach path and landing site. The site had no large hills, high cliffs, or deep craters that could reflect erroneous altitude radar returns to the lunar module landing radar. As with the Apollo 11 landing site, the Ocean of Storms presented a fairly featureless target area. NASA still wanted the least expenditure of spacecraft propellants, with crew and mission safety in mind.
These criteria dictated landing in a mare region near the equator. Mare regions in Ocean of Storms were given high priority because telescopic study suggested that these areas are younger and of a slightly different composition than the Apollo 11 landing site.
Since Apollo 11 overshot its planned target by 4 miles, the goal of Apollo 12 was to demonstrate a precision landing capability. Precise landings capability was vital to set the stage for more complex missions. Therefore, a landing near where Surveyor 3 landed was planned. This provided both a clear marker for determining the accuracy of the landing, and an opportunity to return parts of the Surveyor 3 that had failed to function 2 1/2 years earlier. Of scientific interest was sampling ejecta from the crater Copernicus, in an effort to establish the crater’s age. And, this landing site allowed the CM Yankee Clipper good orbital imaging of the Fra Mauro site that was the target of the ill-fated Apollo 13 mission, and was eventually achieved by the Apollo 14 mission.
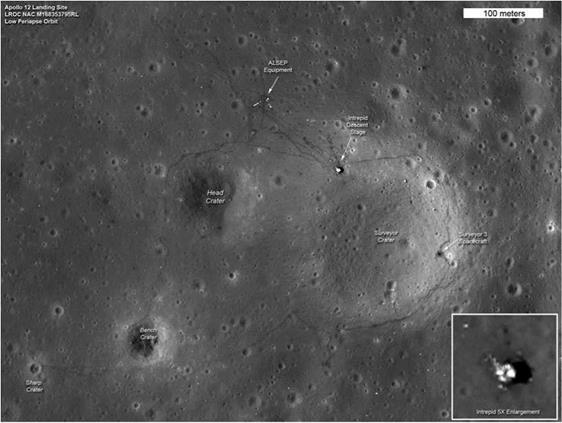
Fig. 4.6 LRO view of Apollo 12 Landing site in 2009. Photo courtesy of NASA and Arizona State University
|
|
 |
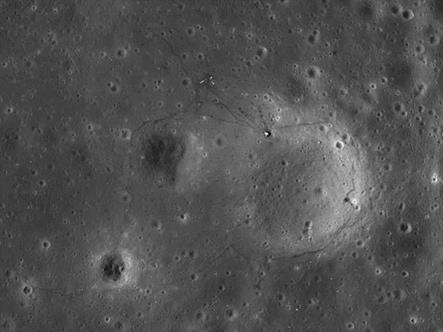
Fig. 4.7 LROviewidentifyingApollo 12. PhotocourtesyofNASAandArizonaStateUniversity
|
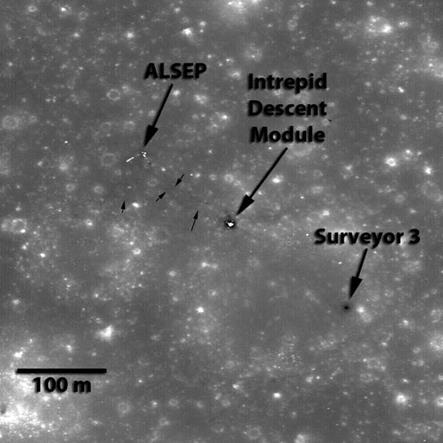
Fig. 4.8 Closer LRO view of the Apollo 12 landing site, an easy neighborhood moon walk away to the Surveyor 3 landing site. Photo courtesy of NASA and Arizona State University
|
As previously mentioned, the difficulty of attempting such a precision landing was complicated by the existence of mass concentrations, or mascons, on the Moon. These mascons caused variations in local gravity on the Moon, variations that easily affected the navigation landing computer onboard the LM, as well as the lunar orbiting CM. These mascon regions were first discovered in 1968, and proved to be a problem for both the American Lunar Orbiter missions, and the Russian Luna missions. NASA’s Lunar Orbiter spacecraft was used to test the Apollo navigation algorithms, and produced errors ten times the specification requirement because of the lunar mascons. Instead of specification error of 200 meters, an error of 2,000 meters would result. An error of almost 1-1/4 mile was intolerable.
NASA had to develop a method to compensate for the mascon error, without actually knowing what a mascon was. The answer was using the Doppler effect to map and compensate for the mascon gravity variations. The Doppler shift of an orbiting LM, although variable, would be predictable. By comparing the predicted Doppler shift against actual Doppler shifts, it could be determined if the LM was off course and how far off course. The trick was how to input the correction information into the LM guidance computer, recognizing the state-of-the-art of the 1960s. The Apollo CM and LM computers were highly primitive by today’s standards. It’s hard to believe that the computer technology of the 1960s limited the LM memory to 2,056 bytes (that’s right, kilobytes, not megabytes, not gigabytes). The technological slight-of-hand was used to fool the computer into thinking the landing site had moved instead of the LM being off-course! The result of this software finesse was the Apollo 12 landed within 163 m of the planned target, and an easy lunar stroll to the Surveyor 3 spacecraft.
Once on the Moon, a major task for the crew of Apollo 12 was the collection of lunar soil and rock samples. A major find of Apollo 12 was the discovery of the first KREEP samples. Unique to the Moon’s geology in the highlands, and not found on Earth, KREEP is a moon rock containing potassium (chemical symbol K), rare-earth elements (REE), and phosphorus (chemical symbol P). Containing potassium, phosphorus oxides, rubidium, and lanthanum, KREEP is made up of normally incompatible elements, and was first found in the Apollo 12 samples in agglutinate glasses and polymict breccias.
KREEP and other anorthosite samples represent part of the evidence for the formation of the Moon. The leading theory for the Moon’s formation is that it was formed from the remains of an ancient impact between the Earth and a Mars-sized proto-planetary body about 4.5 billion years ago. This collision threw a large amount of material into orbit around the Earth that eventually became the Moon.
A large amount of energy was generated by this collision. Scientists have deduced that a large portion of the Moon would have liquified, initially creating a magma covered Moon. As the magma crystalized, minerals precipitated and sank to the bottom to form the lunar mantle.
As the Moon solidified, low density anorthosite material began to crystallize and float towards the surface, forming a solid crust. Elements that are usually incompatible then progressively concentrate into the magma. The magma that formed, sandwiched between the crust and mantle, was rich in KREEP. The evidence for these processes came from the presence of the rocks rich in KREEP, and the highly anorthositic composition of the crust of the lunar highlands from samples from later Apollo missions.
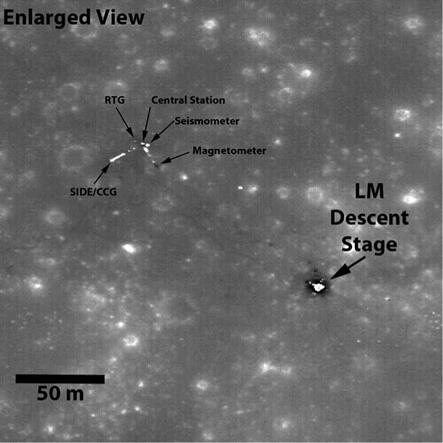
Fig. 4.9 LRO view of Apollo 12 ALSEP, clearly showing the experiments Astronauts Bean and Conrad deployed as part of the Geophysical Station. Photo courtesy of NASA and Arizona State University
|
The Apollo Lunar Surface Experiments Package, or ALSEP, aboard Apollo 12 and deployed by Astronauts Conrad and Bean at the Ocean of Storms was made up of a more extensive set of experiments than the EASEP of Apollo 11:
• The Passive Seismic Experiment (PSE) detected lunar seismic activities and provided information about the internal structure of the Moon. The Passive Seismic Experiment studied the propagation of seismic waves through the Moon and provided data about the Moon’s internal structure. The Apollo 11 seismometer returned data for just 3 weeks but provided a useful first look at lunar seismology. More advanced seismometers were deployed at the Apollo 12, 14, 15, and 16 landing sites and transmitted data to Earth until September 1977.
Each of the PSE’s measured all three components of ground displacement (up-down, north-south, and east-west). In 2011, based on 30 year old data from these four PSE sites, a new analysis confirmed the layering of the Moon’s core. This analysis, using a technique borrowed from one originally developed to analyze Earth’s earthquakes, concludes that the Moon’s core has a solid inner core surrounded by a molten outer core. Additionally, the analysis suggested that the iron-rich lunar inner core contains less than 6 % of the light elements of the Periodic Table. Most useful for this study utilized data from more than 6,000 deep moonquakes occurring about 435 miles below the Moon’s surface.
• The Lunar Surface Magnetometer (LSM) measured the strength of the Moon’s magnetic field. The magnetic field data included field strengths both from the Moon’s intrinsic magnetic field mixed with external magnetic fields of the Earth and Sun. These external fields varied in strength with time as the Moon moved through Earth’s magnetosphere as it orbited the Earth. By making measurements over several months, these time-varying fields were be separated from the Moon’s steady, intrinsic magnetic field. LSM experiments were part the ALSEP included in the Apollo 12, 15, and 16 missions.
• The Cold Cathode Gauge (CCG) measured the atmospheric pressure of the lunar atmosphere. On Apollo 12, the CCG instrumentation was a part of the Supra Ion Detector Experiment (SIDE). Unfortunately, the CCG failed after 14 hours. The strong magnetic field of the CCG caused interference with the SIDE, which resulted the experiment was packaged as a standalone implementation in later missions.
• The Suprathermal Ion Detector Experiment (SIDE) studied the lunar ionosphere, by measuring the various properties of positive ions in the Lunar environment, collecting data on the plasma interaction between solar wind and the Moon, and measuring the electrical potential of the Lunar surface.
• The Solar Wind Spectrometer (SWS), in addition to the SWCE, was identical to that included in the Apollo 11 EASEP, measured the composition of the solar wind. This experiment, unlike the SWCE, continually sampled the solar wind to measure the effects of the Earth’s magnetic field on the constitution of the solar wind. The results of this experiment showed that most of the Moon’s orbit, which is outside the Earth’s magnetic field, a proton density of 10-20 protons per cubic centimeter was detected. However, no protons were detected during the 5 days in which the Moon is in the tail of the Earth’s magnetic field. Clearly, the SWS demonstrated the shielding effect of the Earth’s magnetic field from the solar wind.
• The Lunar Dust Detector (LDD) studied the effects of lunar dust on the operation of the experiment package.
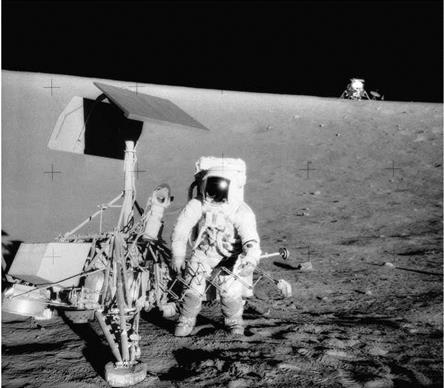
Fig. 4.10 hoto of Astronaut Conrad and Surveyor 3. Courtesy of NASA
|
Pete Conrad Jr., Apollo 12 Commander, examined the unmanned Surveyor III spacecraft during the second moon walk as seen in Fig. 4.10. The “Intrepid” landed on the Moon’s Ocean of Storms less than 600 feet from Surveyor 3. The television camera and several other components were taken from Surveyor 3 and brought back to earth for scientific analysis. Surveyor 3 soft-landed on the Moon on 19 April, 1967.
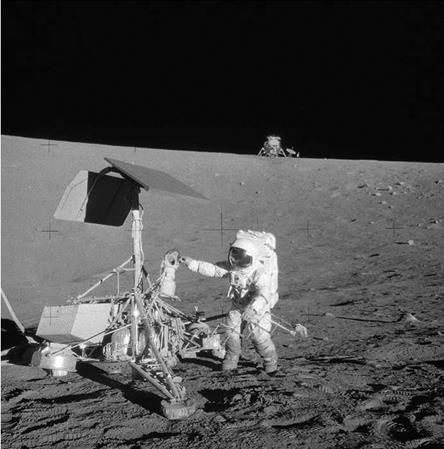
Fig. 4.11 Surveyor 3. Courtesy of NASA
|
Surveyor 3 was unintentionally the first spacecraft to liftoff from the Moon’s surface. In fact, it did this twice. Due to a problem with the Surveyor 3 landing radar, which did not shut off the vernier engines, kept them firing throughout the first touchdown, and continued until a second touchdown. The Lunar Module Intrepid landed 600 feet from Surveyor 3, as planned. Surveyor 3’s TV and telemetry systems were found to have been damaged by its unplanned landings and liftoffs.
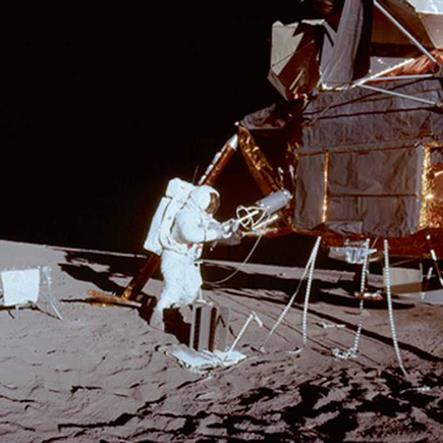
Fig. 4.12 Astronaut Alan Bean during experiment setup. Courtesy of NASA
|
Another element of Apollo 12 is the Radioactive Thermoelectric Generator. Astronaut Alan Bean can be seen in Fig. 4.12 unloading the nuclear fuel element for the RTG in the foreground. Bean is attempting to remove the fuel element from the cask using the Fuel Transfer Tool (FTT). On Apollo 12, the fuel element stuck in the cask because of thermal expansion (Bean could feel the heat through his suit). Conrad pounded the side of the cask with a hammer while Bean successfully worked it loose. He then inserted it into the RTG and discarded the FTT.
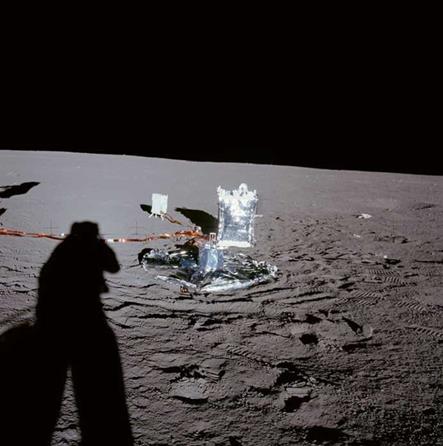
Fig. 4.13 Apollo Lunar Surface Experiments Package (the ALSEP portable scientific lab). Courtesy of NASA
|
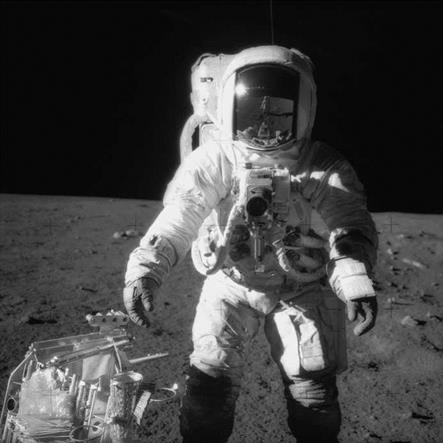
Fig. 4.14 LM Pilot and future artist Alan Bean. Alan Bean has produced a number of paintings and published a book of his artwork depicting his and NASA’s moon experiences. A select number of his paintings actually contain moon dust mixed into the paints. Courtesy of NASA
|
From a public relations point-of-view, the only part of the mission that had gone really wrong was the failure of the color TV camera. The public had great expectations for a spectacular television broadcast from the Moon. The Apollo 11 television camera produced low resolution, grainy, black-and-white video. This was the first color TV camera to be used on the Moon. Unfortunately, the development of the camera had experienced delays, and the contractor Westinghouse delivered the camera to NASA just days before the launch. As a workaround, Alan Bean trained with a mockup of the camera, which did not highlight the design limitations of the equipment. Initially, the TV camera was operational, but 42 minutes into telecasting the first EVA, astronaut Bean accidentally pointed the camera at the Sun while preparing to mount it on the tripod. The Sun’s extreme brightness burned out the vidicon imaging tube, rendering the camera inoperable. When the camera was returned to Earth, it was shipped to Westinghouse for post-mission analysis. Westinghouse engineers were able to get an image on the section of the tube that wasn’t damaged. Procedures for future Apollo missions were re-written in order to prevent such damage, including the addition of a lens cap to protect the tube when the camera was repositioned. Fortunately, the loss of the TV camera was not crucial from a technical point of view, and did not impact the success of the mission. However, the loss of television coverage of the Apollo 12 mission disappointed millions of viewers in the United States and the world.
In the early Apollo lunar missions, the Saturn V S-IVB third stage that propelled the CSM/LM towards the Moon during the trans-lunar injection phase was lost in space and allowed to enter a heliocentric orbit following separation. Apollo 13, 14, 15, 16, and 17 used the spent S-IVB stage as an opportunity to perform seismic studies and were deliberately crashed into the Moon. The third stage impact provided seismic data to the ALSEP seismometers.
The Apollo 12 Saturn third stage did not impact the Moon, but was supposed to be sent into heliocentric orbit, and was considered lost. Then on September 3, 2002, an amateur astronomer named Bill Yeung discovered what was then considered an asteroid, and officially given the designation of J002E3. At the time of its discovery, J002E3 was in a highly elliptical orbit around Earth. University of Arizona astronomers found that the object’s spectrum was consistent with titanium dioxide paint, the type of paint used on the Saturn V launch vehicles. An analysis of the orbit has identified the Apollo 12 third stage as the likely candidate for J002E3. The Apollo 12 S-IVB did not achieve a true heliocentric orbit, having not achieving escape velocity for the Earth-Moon system. After flying past the Moon on November 18, 1969, J002E3 attained a semi-stable orbit in which the third stage entered a highly elliptical orbit around the Earth-Moon system, then flung into deep space by the combined gravity forces of Earth and the Moon, and then re-captured by the Earth – Moon system at a later date. Following its discovery, J002E3 left Earth orbit in 2003, and is expected to return in either 2032, or the mid-2040s, depending on the orbital model used.
The mystery of the mascons has finally been solved. In May, 2013, NASA released the findings of the Gravity Recovery and Interior Laboratory (GRAIL) mission to the Moon. GRAIL consisted of two lunar orbiting probes, GRAIL A and GRAIL B, launched in September 2011, orbiting in near circular orbits at an altitude of 55 miles. The distance between the two GRAIL probes, nicknamed Ebb and Flow, were precisely measured as they passed over gravity variations caused by mountains, craters and mascons until the data collection ended in December of 2012. NASA reported that the GRAIL data confirmed the lunar mascons were generated by large asteroids and comets impacting the young Moon when it had a thin crust and dense molten mantle. The impact created a circular ripple effect of material that cooled beneath the visible surface of the Moon consisting of rings of dense and lighter lunar material.