News of Rontgen’s discovery of X-rays rippled around the world. “When Rontgen discovered X-rays in November of 1895 by placing his hand between a Crookes Tube and a florescent screen, the medical applications of the technology were immediately appar – ent.”4 By January 1896, Rontgen had issued a report regarding his experiments to media in Europe and the United States. The public was immediately fascinated by the possibility of seeing through seemingly solid objects or inside the human body, and the topic made headlines in newspapers and weeklies. Apparently, X-r ay
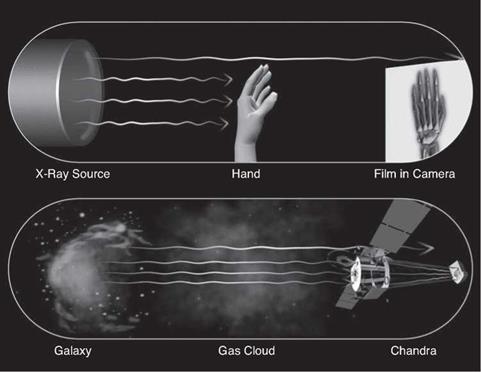
Figure 10.1. At the end of the nineteenth century, X-rays were harnessed for medical imaging, where dense material like bones cast a shadow in X-rays. In the astronomical realm, a very hot gas or plasma emits X-rays, as do regions near compact objects like black holes. The X-rays may also be absorbed by intervening material, and they can only be detected by a satellite above the Earth’s atmosphere (NASA/CXC/M. Weiss).
|
“mania” swept through the public sphere but at a level far surpassing a simple cultural fad, explains Nancy Knight, who notes that “X-rays appeared in advertising, songs, and cartoons.”5 John Lienhard similarly reports, “Seldom has anything taken hold of the public imagination so powerfully and completely. . . . Right away, magazine cartoons celebrated the idea. A typical one showed a man using an X-ray viewer to see through a lady’s hat at the theatre.”6
Edwin Gerson has investigated the speed with which X-rays were incorporated into the branding of products in the United States, and he explores why so many marketers used the term X – ray to appeal to consumers. Being a medical doctor, Gerson began collecting household products dating back to the 1890s, none of which have anything whatsoever to do with X-rays. One could purchase Sniteman’s X-Ray Liniment for horses and cattle, and Patt’s X-Ray Liniment for people. X-Ray Golf Balls, ostensibly to improve the accuracy of one’s game, were sold by John Wana – maker of New York. There was the D-cell X-Ray flashlight battery, X-Ray Stove Polish, X-Ray Cream Furniture Polish manufactured in New York, and X-Ray Soap made in Port Huron, Michigan. X-Ray Blue Double Edge razor blades were touted as “the finest blade known to science,” while drinking fountains for chicken houses were marketed by the X-Ray Incubator Company in Wayne, Nebraska. Housewives could purchase an X-ray Coffee Grinder or the X-Ray lemon squeezer, and the X-Ray Raisin Seeder was available as early as November 1896. A company from Baltimore, Maryland, sold boxes of X-Ray headache tablets, with 8 pills for ten cents promising relief in just fifteen minutes.7 Clearly, advertising by means of non sequitur is not just a phenomenon of the modern age.
The popular fascination with X-rays continued for decades. By 1924, an X-ray shoe fitter was deployed by shoe vendors to image both feet inside a new pair of shoes. David Lapp reports that in the United States “there were approximately 10,000 of these fluo – roscopes in use, being made by companies such as Adrian X-Ray Shoe Fitter,” and that by the early 1950s most shoe stores were using the devices.8 As Gerson points out, “The public was simply astonished with X rays, and advertisers played off this spellbound attention by adding the name to almost any type of product. . . . Not only did the image of the X ray convey a sense of cutting-edge technology, it also functioned as a metaphor for ‘powerful unseen truth and strength.’”9
Researchers and medical personnel working with X-rays were frequently exposed to dangerous or fatal levels of radiation, including Elizabeth Fleischmann, who at age 28 read a news article on how to build an X-ray device and by 1900 became the best medical radiologist in the United States. But in ten years’ time, Fleischmann had been so badly overexposed to radiation by imaging her patients and developing the films that she did not sur – vive.10 At the time, the effect of exposure to radiation was poorly understood and this ignorance played out in dangerous exposure for both medical practitioners and those working with the new technology for purposes of entertainment. Nancy Knight, for instance, indicates that “papers reported daily” on Thomas Edison’s efforts to X-ray a person’s brain in order to figure out how the brain functions.11
Allen Grove reports that some speculated at the time that the new technology might afford a kind of X-ray vision that might make it possible to capture on film the images of ghosts, apparently a popular notion in England. He also comments that within months of Rontgen’s announcement, theaters were headlining performances inspired by the popularity of X-rays.12 Piano sheet music for American composer Harry L. Tyler’s “X Ray Waltzes” from 1896 depicted on its cover a man holding his hand under an X-ray tube. Beneath the man’s hand appears a skeletal X-ray image. In fact, in 2010, Tyler’s waltzes were dusted off and featured in a BBC broadcast titled “Images that Changed the World.” And, any discussion of X-rays in popular culture must recall that in the 1930s, when writer Jerry Siegel and artist Joe Shuster invented the prototypical superhero, it was inevitable that Superman would be gifted with X-ray vision. Though Superman didn’t have such ability at the outset, in later adventures he is able to see in multiple wavelengths of the electromagnetic spectrum, including radio waves, infrared, ultraviolet, and eventually X-rays.13
Rontgen’s discovery had a deep shaping effect on the arts. This was because X-rays were an entree into a hidden world, the “world within the world” represented by the interior structure of atoms and molecules. In 1912, less than twenty years after the initial discovery, physicist Max von Laue showed that X-rays were a form of electromagnetic radiation because they diffracted when interacting with matter. A year later, the father and son team of William Henry and William Lawrence Bragg described mathematically how X-rays scattered within a crystal, winning the Nobel Prize in Physics for their work in 1915. This opened the door to X-ray crystallography, a powerful tool for measuring the spacing and arrangement of atoms within a solid. The impact of this discovery was particularly strong in the visual arts, which were undergoing their own revolution with the onset of Fauvism, Cubism, and Futurism. The X-ray was liberating because to artists it “proved that the external is not valid, it’s just a false layer.”14
Art historian Linda Dalrymple Henderson contends that X-rays suggested the possibility of stepping into the fourth dimension. She writes, “From the 1880’s to the 1920’s, popular fascination with an invisible, higher dimension of space—of which our familiar world might be only a section or shadow—is readily apparent in the vast number of articles and the books. . . published on the topic.” Henderson has demonstrated that modern artists readily embraced X-rays, and their ability to expose realities and perspectives not perceptible to the human eye, as suggestive of fourdimensional space.15 Cubist paintings, with their simultaneous depiction of all sides of a seemingly transparent object, and their suggestion of interior planes, explains Henderson, “are testaments to the new paradigm of reality ushered in by the discovery of X – rays and interest in the fourth dimension. Such paintings are new kinds of ‘windows’—in this case, into the complex, invisible reality or higher dimensional world as imagined by the artist.”16 The inspiration was sometimes very direct. According to historian Arthur Miller, “Picasso’s Standing Female Nude” (1910) was inspired by the power of X-rays to glimpse beyond the visible: what you see is not what you get. In this case, the inspiration was X-ray photographs taken to diagnose the illness of Picasso’s mistress, Fernande Olivier. Superposed on a background of planes, her body lies open to reveal pelvic hip bones made up of geometrical shapes: forms reduced to geometry—the aesthetic of Cubism, inspired by modern science.”17 From the arts, to medicine, and particularly through astronomy and space exploration, X-rays have profoundly altered human culture.