The systematic sonic boom testing that NASA began in 1958 would exponentially expand the heretofore largely theoretical and anecdotal knowledge about sonic booms with a vast amount of "real world” data. The new information would make possible increasingly sophisticated experiments and provide feedback for checking and refining theories and mathematical models. Because of the priority bestowed on sonic boom research by the SST program and the numerous types of aircraft then available for creating booms (including some faster than anything flying today), the data and findings from the tests conducted in the 1960s are still of significant value in the 21st century.[351]
The Langley Research Center (often referred to as NASA Langley) served as the Agency’s "team leader” for supersonic research. Langley’s acoustics specialists conducted NASA’s initial sonic boom tests in 1958 and 1959 at the Wallops Island Station on Virginia’s isolated Delmarva Peninsula. During the first year, they used six sorties by NASA F-100 and F-101 fighters, flying at speeds between Mach 1.1 and 1.4 and altitudes from 25,000 to 45,000 feet, to make the first good ground recordings and measurements of sonic booms for steady, level flights (the kind of profile a future airliner would fly). Observers judged some of the booms above
1.0 psf to be objectionable, likening them to nearby thunder, and a sample plate glass window was cracked by one plane flying at 25,000 feet. The 1959 test measured shock waves from 26 flights of a Chance Vought F8U-3 (a highly advanced prototype based on the Navy’s Crusader fighter) at speeds up to Mach 2 and altitudes up to 60,000 feet. A B-58 from Edwards AFB also made two supersonic passes at 41,000 feet. Boom intensities from these higher altitudes seemed to be tolerable to observers, with negligible increases in measured overpressures between Mach 1.4 and 2.0. These results were, however, very preliminary.[352]
In July 1960, NASA and the Air Force conducted Project Little Boom at a bombing range north of Nellis AFB, NV, to measure the effects on structures and people of extremely powerful sonic booms. F-104 and F-105 fighters flew slightly over the speed of sound (Mach 1.09 to 1.2) at altitudes as low as 50 feet above ground level. There were more than 50 incidents of sample windows being broken at 20 to 100 psf, but only a few possible breakages below 20 psf, and no physical or psychological harm to volunteers exposed to overpressures as high as 120 psf.[353] At Indian Springs, Air Force fighters flew supersonically over an instrumented C-47 transport from Edwards, both in the process of landing and on the ground. Despite 120 psf overpressures, there was only very minor damage when on the ground and no problems in flight.[354] Air Force fighters once again would test powerful sonic booms in 1965 in support of Joint Task Force 2 at Tonopah, NV. The strongest sonic boom ever recorded, 144 psf, was generated by an Air Force F-4E Phantom II flying Mach 1.26 at 95 feet.[355]
In late 1960 and early 1961, NASA and AFFTC followed up on Little Boom with Project Big Boom. B-58 bombers made 16 passes flying Mach 1.5 at altitudes of 30,000 to 50,000 feet over arrays of sensors, which measured a maximum overpressure of 2.1 psf. Varying the bomber’s weight from 82,000 to 120,000 pounds provided the first hard data on how an aircraft’s weight and related lift produced higher over-pressures than existing theories based on volume alone would indicate.[356]
Throughout the 1960s, Edwards Air Force Base—with its unequaled combination of Air Force and NASA expertise, facilities, instrumentation, airspace, emergency landing space, and types of aircraft—hosted the largest number of sonic boom tests. NASA researchers from Langley’s Acoustics Division spent much of their time there working with the Flight Research Center in a wide variety of flight experiments. The Air Force Flight Test Center usually participated as well.
In an early test in 1961, Gareth Jordan of the FRC led an effort to collect measurements from F-104s and B-58s flying at speeds of Mach 1.2 to 2.0 over sensors located along Edward AFB’s supersonic corridor and at Air Force Plant 42 in Palmdale, about 20 miles south. Most of the Palmdale measurements were under 1.0 psf, which the vast majority of people surveyed there and in Lancaster (where overpressures tended to be somewhat higher) considered no worse than distant thunder. But there were some exceptions.[357]
Other experiments at Edwards in 1961 conducted by Langley personnel with support from the FRC and AFFTC contributed a variety of new information. With help from a tethered balloon, they made the first good measurements of atmospheric effects, showing that air turbulence in the lower atmosphere (known as the boundary layer) significantly affected wave shape and overpressure. They also gathered the first data on booms from very high altitudes. Using an aggressive flight profile, AFFTC’s B-58 crew managed to zoom up to 75,000 feet—25,000 feet higher than the bomber’s normal cruising altitude and 15,000 feet over its design limit! The overpressures measured from this high altitude proved stronger than predicted (not a promising result for the planned SST). Much lower down, fighter aircraft performed accelerating and turning maneuvers to generate the kind of acoustical rays that amplified shock waves and produced multiple booms and super booms. Thevarious experiments showed that a combination of atmospheric conditions, altitude, speed, flight path, aircraft configuration, and sensor location determined the shape of the pressure signatures.[358]
Of major significance for future boom minimization efforts, NASA also began making in-flight shock wave measurements. The first of these, at Edwards in 1960, had used an F-100 with a sensor probe to measure supersonic shock waves from the sides of an F-100, F-104, and B-58, as well as from F-100s speeding past with only 100 feet of separation. The data confirmed Whitham’s overall theory, with some discrepancies. In early 1963, an F-106 equipped with a sophisticated new sensor probe designed at Langley flew seven sorties both above and below a B-58 at speeds of Mach 1.42 to 1.69 and altitudes of approximately 40,000 to
50,0 feet. The data gathered confirmed Walkden’s theory that lift as well as volume increases peak shock wave pressures. As indicated by
Figure 3, analysis of the readings also found that the bow and tail shock waves spread farther apart as they flowed from the B-58 and showed how the multiple or "saw tooth” shock waves produced by the rest of an airplane’s structure (e. g., fuselage, canopy, wings, engines, nacelles, etc.) merged with the stronger bow and tail waves until—at a distance of between 50 and 90 body lengths—they began to coalesce into the classic N-shaped signature.[359] This marked a major milestone in sonic boom research.
One of the most publicized and extended flight test programs at Edwards had begun in 1959 with the first launch from a B-52 of the fastest aircraft ever flown: the rocket-propelled X-15. T hree of these legendary aerospace vehicles expanded the envelope and gathered data on supersonic and hypersonic flight for the next 8 years. Although the X-15 was not specifically dedicated to sonic boom tests, the Flight Research Center did begin placing microphones and tape recorders under the X-15s’ flight tracks in the fall of 1961 to gather boom data. FRC researchers much later reported on the measurements of these sonic booms, made at speeds of Mach 3.5 and Mach 4.8.[360]
For the first few years, NASA’s sonic boom tests occurred in relative isolation within military airspace in the desert Southwest or over Virginia’s rural Eastern Shore. A future SST, however, would have to fly over heavily populated areas. Thus, from July 1961 through January 1962, NASA, the FAA, and the Air Force carried out the Community and Structural Response Program at St. Louis, MO. In Operation Bongo, the Air Force sent B-58 bombers on 76 supersonic training flights over the city at altitudes from 31,000 to 41,000 feet, announcing them as routine SAC radar bomb-scoring missions. F-106 interceptors flew 11 additional flights at 41,000 feet. Langley personnel installed sensors on the ground, which measured overpressures up to 3.1 psf. Investigators from Scott AFB, IL, or for a short time, a NASA-contracted engineering firm, responded to dam-
 |
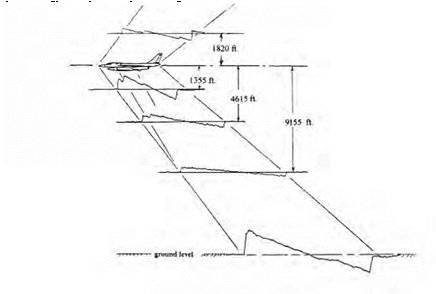 |
age claims, finding some possibly legitimate minor damage in about 20 percent of the cases. Repeated interviews with more than 1,000 residents found 90 percent were at least somewhat affected by the booms and about 35 percent were annoyed. Scott AFB (a long-distance phone call from St. Louis) received about 3,000 complaints during the test and another 2,000 in response to 74 sonic booms in the following 3 months. The Air Force eventually approved 825 claims for $58,648. These results served as a warning that repeated sonic booms could pose an issue for SST operations.[361]
To obtain more definitive data on structural damage, NASA in December 1962 resumed tests at Wallops Island using various sample buildings. Air Force F-104s and B-58s and Navy F4H Phantom IIs flew at altitudes from 32,000 to 62,000 feet, creating overpressures up to3 psf. Results indicated that cracks to plaster, tile, and other brittle materials triggered by sonic booms occurred in spots where the materials were already under stress (a finding that would be repeated in later more comprehensive tests).[362]
In February 1963, NASA, the FAA, and the USAF conducted Project Littleman at Edwards AFB to see what happened when two specially instrumented light aircraft were subjected to sonic booms. F-104s made 23 supersonic passes at distances as near as 560 feet from a little Piper Colt and a 2-engine Beech C-45, creating overpressures up to 16 psf. Their responses were "so small as to be insignificant”—dismissing one possible concern about SST operations.[363]
The St. Louis survey had left many unanswered questions on public opinion. To learn more, the FAA’s Supersonic Transport Development Office, with support from NASA Langley and the USAF (including Tinker AFB), next conducted the Oklahoma City Public Reaction Study from February through July 1964. This was a much more intensive and systematic test. In an operation named Bongo II, B-58s, F-104s, F-101s, and F-106s were called upon to deliver sonic booms between 1.0 and
2.0 psf, 8 times per day, 7 days a week, for 26 weeks, with another 13 weeks of followup activities. The aircraft flew a total of 1,253 supersonic flights at Mach 1.2 to 2.0 and altitudes between 21,000 and 50,000 feet.
The FAA (which had a major field organization in Oklahoma City) instrumented nine control houses scattered throughout the metropolitan area with various sensors to measure structural effects, while experts from Langley instrumented three houses and set up additional sensors throughout the area to record overpressures, wave patterns, and meteorological conditions. The National Opinion Research Center at the University of Chicago interviewed a sample of 3,000 adults three times during the study. By the end of the test, 73 percent of those surveyed felt that they could live with the number and strength of the booms experienced, and 27 percent would not accept indefinite booms at the level tested. Forty percent believed that they caused some structural damage (even though the control houses showed no significant effects). Analysis of the shock wave patterns by NASA Langley showed that a small number of overpressure measurements were significantly higher than expected, indicating probable atmospheric influences, including heat rising from urban landscapes. One possible result was the breakage of almost 150 windows in the city’s two tallest buildings early in the test.[364]
The Oklahoma City study added to the growing knowledge about sonic booms and their acceptance by the public at the cost of negative publicity for the FAA. In view of the reactions to the St Louis and Oklahoma City tests by much of the public and some politicians, plans for another extended sonic boom test over a different city, including flights at night, never materialized.[365]
The FAA and Air Force conducted the next series of tests from November 1964 to February 1965 in a much less populated place: the remote Oscura camp in the vast White Sands Missile Range of New Mexico, where 21 structures of various types and ages with a variety of plaster, windows, and furnishings were studied for possible damage. F-104s from nearby Holloman AFB and B-58s from Edwards generated 1,494 booms producing overpressures from 1.6 to 19 psf. The 680 sonic booms at 5.0 psf caused no real problems, but those above 7.9 psf revealed varying degrees of damage to glass, plaster, tile, and stucco already in vulnerable condition. A parallel study of several thousand incubated chicken eggs showed no reduction in hatchabil – ity, and audiology tests on 20 personnel subjected daily to the booms showed no hearing impairment.[366]
Before the White Sands test ended, NASA Langley personnel began collecting boom data from a highly urbanized setting in winter weather. During February and March 1965, they recorded data at five ground stations as B-58 bombers flew 22 training missions in a corridor over downtown Chicago at speeds of Mach 1.2 to 1.66 and altitudes from
38,0 to 48,000 feet. The results showed how amplitude and wave shape varied widely depending upon atmospheric conditions. These 22 flights and 27 others resulted in the Air Force approving 1,442 of 2,964 damage claims for $114,763.[367]
Also in March 1965, the FAA and NASA, in cooperation with the
U. S. Forest Service, studied the effect on hazardous mountain snow packs in the Colorado Rockies of Air Force fighters creating boom overpressures up to 5.0 psf. Because of stable snow conditions, none of these created an avalanche. Interestingly enough, in the early 1960s the
National Park Service had tried to use newly deployed F-106s at Geiger Field, WA, to create controlled avalanches in Glacier National Park (Project "Safe Slide”), but presumably found traditional artillery fire more suitable.[368]
From the beginning of the SST program, the aircraft most desired for experiments was, of course, the North American XB-70 Valkyrie. The first of the giant testbeds (XB-70-1) arrived at Edwards AFB in September 1964, and the better performing and better instrumented second aircraft (XB-70-2) arrived in July 1965. With a length of 186 feet, a wingspan of 105 feet, and a gross weight of about 500,000 pounds, the six-engine giant was less than two-thirds as long as some of the later SST concepts, but it was the best real-life surrogate available.
Even during the initial flight envelope expansion by contractor and AFFTC test pilots, the Flight Research Center began gathering sonic boom data, including direct comparisons of its shock waves with those of a B-58 flying only 800 feet behind.[369] Using an array of microphones and recording equipment at several ground stations, NASA researchers eventually built a database of boom signatures from 39 flights made by the XB-70s (10 with B-58 chase planes), from March 1965 through May 1966.[370] Because "the XB-70 is capable of duplicating the SST flight profiles and environment in almost every respect,” the FRC was looking forward to beginning its own experimental research program using the second Valkyrie on June 15, 1966, with sonic boom testing listed as the first priority.[371]
On June 8, however, XB-70-2 crashed on its 47th flight as the result of an infamous midair collision that killed two pilots and gravely injured
a third. Despite this tragic setback to the test program, the less capable XB-70-1 (which underwent modifications until November) eventually proved useful for many purposes. After 6 months of joint AFFTC/FRC operations, including the boom testing described below, the plane was turned over full time to NASA in April 1967 after 60 Air Force flights. The FRC, with a more limited budget, then used the Valkyrie for 23 more test missions until February 1969, when the unique aircraft was retired to the USAF Museum in Dayton, OH.[372] All told, NASA acquired sonic boom measurements from 51 of the 129 total flights made by the XB-70s, using two ground stations on Edwards AFB, one at nearby Boron, and two in Nevada.[373] This data proved to be of great value in the future.
The loss of one XB-70 and retirement of the other from supersonic testing was made somewhat less painful by the availability of another smaller but even faster product of advanced aviation technology: the Lockheed YF-12 and its cousin, the SR-71—both nicknamed Blackbirds. On May 1, 1965, shortly after arriving at Edwards, a YF-12A set nine world records, including a closed-course speed of 2,070 mph (Mach 3.14)
and a sustained altitude of 80,257 feet. Four of that day’s five flights also yielded sonic boom measurements. At speeds of Mach 2.6 to 3.1 and altitudes of 60,000 to 76,500 feet above ground level, overpressures varied from 1.2 to 1.7 psf depending on distance from the flight path. During another series of flight tests at slower speeds and lower altitudes, overpressures up to 5.0 psf were measured during accelerations after having slowed to refuel. These early results proved consistent with previous B-58 data.[374] Data gathered over the years from ground arrays measuring the sonic signatures from YF-12s, XB-70s, B-58s, and smaller aircraft flying at various altitudes also showed that the lateral spread of a boom carpet (without the influence of atmospheric variables) could be roughly equated to 1 mile for every 1,000 feet of altitude, with the N-signatures become more rounded with distance until degenerating into the approximate shape of a sine wave.[375]
Although grateful to benefit from the flights of AFFTC’s Blackbirds, the FRC wanted its own YF-12 or SR-71 for supersonic research. It finally gained the use of two YF-12s through a NASA-USAF memorandum of understanding signed in June 1969, paying for operations with funding left over from termination of the X-15 and XB-70 programs.[376]
In the fall of 1965, with public acceptance of sonic booms becoming a significant public and political issue, the White House Office of Science and Technology established the National Sonic Boom Evaluation Office (NSBEO) under an interagency Coordinating Committee on Sonic Boom Studies. The new organization, which was attached to Air Force Headquarters for administrative purposes, planned a comprehensive series of tests known as the National Sonic Boom Evaluation Program, to be conducted primarily at Edwards AFB. NASA (in particular the Flight Research and Langley Centers) would be responsible for test operations and data collection, with the Stanford Research Institute hired to help analyze the findings.[377] After careful preparations (including specially built structures and extensive sensor and recording arrays), the National Sonic Boom Evaluation began in June 1966. Its main objectives were to address the many issues left unresolved from previous tests. Unfortunately, the loss of XB-70-2 on June 8 forced a 4-month break in the test schedule, with the limited events completed in June designated Phase I. The second phase began in November, when XB-70-1 returned to flight status, and lasted into January 1967. A total of 367 supersonic missions were flown by XB-70s, B-58s, YF-12s, SR-71s, F-104s, and F-106s during the two phases. These were supplemented by 256 subsonic flights by KC-135s, WC-135Bs, C-131Bs, and Cessna 150s. In addition, the Goodyear blimp Mayflower was used in the June phase to measure sonic booms at 2,000 feet.[378]
By the end of testing, the National Sonic Boom Evaluation had obtained new and highly detailed acoustic and seismic signatures from all the different supersonic aircraft in various flight profiles during a variety of atmospheric conditions. The data from 20 XB-70 flights at speeds from Mach 1.38 to 2.94 were to be of particular long-term interest. For example, Langley’s sophisticated nose probe used for the pioneering in-flight flow-field measurements of the B-58 in 1963 was installed on one of the FRC’s F-104s to do the same for the XB-70. Comparison of data between blimp and ground sensors and variations between the summer and winter tests confirmed the significant influence that atmospheric conditions, such as turbulence and convective heating near the surface, have on boom propagation. [379] Also, the evaluation provided an opportunity to gather data on more than 1,500 sonic boom signatures created during 35 flights by the recently available SR-71s and YF-12s at speeds up to Mach 3.0 and altitudes up to 80,000 feet.[380]
Some of the findings portended serious problems for planned SST operations. The program obtained responses from several hundred volunteers, both outdoors and in houses, to sonic booms of different intensities produced by each of the supersonic aircraft. The time between the peak overpressure of the bow and tail waves for aircraft at high altitudes ranged from about 0.1 second for the F-104, 0.2 second for the B-58, and 0.3 second for the XB-70. The respondents also compared sonic booms to the jet engine noise of subsonic aircraft. Although data varied for each of the criteria measured, significant minorities tended to find the booms either "just acceptable” or unacceptable, with the "sharper” N-wave signature from the lower flying F-104 more annoying outdoors than the more rounded signatures from the larger aircraft, which had to fly at higher altitudes to create the same overpressure. Other factors included the frequency, time of day or night, and type of boom signature. Correlating how the subjects responded to jet noise (measured in decibels) and sonic booms (normally measured in psf), the SRI researchers used the perceived noise decibel (PNdB) level to assess how loud booms seem to human ears.[381]
Employing sophisticated sensors, civil engineers measured the physical effects on houses and a building with a large interior space (the base bowling alley) to varying degrees of booms created by F-104s, B-58s, and the XB-70. Of special concern for the SST, they found the XB-70’s elongated N-wave created more of the low frequencies that cause indoor vibrations, such as rattling windows (although less bothersome to observers outdoors). And although no significant harm was detected to the instrumented structures, 57 complaints of damage were received from residents in the surrounding area, and three windows were broken on base. Finally, monitoring by the Department of Agriculture detected no ill effects on farm animals in the area, although avian species (chickens, turkeys, etc.) reacted more than livestock did.[382] The National Sonic Boom Evaluation remains the most comprehensive such test program yet conducted.
Later, in 1967, the opportunity for collecting additional survey data presented itself when the FAA and NASA learned that SAC was starting an extensive training program for its growing fleet of SR-71s. TRACOR,
Inc., of Austin, TX, which was already under contract to NASA doing surveys on airport noise, had its contract expanded in May 1967 to include public responses to the SR-71s’ sonic booms in Dallas, Los Angeles, Denver, Atlanta, Chicago, and Minneapolis. Between July 3 and October 2, Air Force SR-71s made 220 flights over these cities at high altitude, ranging from 5 over Atlanta to 60 over Dallas. The minority of sonic booms that were measured were almost all N-waves with overpressures from slightly less than 1.0 psf to 2.0 psf. Although the data from this impromptu test program were less than definitive, the overall findings (based on 6,375 interviews) were fairly consistent with the previous human response surveys. For example, after an initial dropoff, the level of annoyance with the booms tended to increase over time, and almost all those who complained were worried about damage. Among 15 different adjectives supplied to describe the booms (e. g., disturbing, annoying, irritating), the word "startling” was chosen much more than any other.[383]
Although the FRC and AFFTC continued their missions of supersonic flight-testing and experimentation at Edwards, what might be called the heroic era of sonic boom testing was drawing to a close. The FAA and the Environmental Science Services Administration (a precursor of the Environmental Protection Agency) did some sophisticated testing of meteorological effects at Pendleton, OR, from September 1968 until May 1970, using a dense grid of recently invented unattended recording equipment to measure random booms from SR-71s. On the other side of the continent, NASA and the Navy studied sonic booms during Apollo missions in 1970 and 1971.[384]
The most significant NASA testing in 1970 took place from August through October at the Atomic Energy Commission’s Jackass Flats test site in Nevada. In conjunction with the FAA and the National Oceanic and Atmospheric Administration (NOAA), NASA took advantage of the 1,527-foot-tall BREN Tower (named for its original purpose, the "Bare Reactor Experiment Nevada” in 1962) to install a vertical array of 15 microphones as well as meteorological sensors. (Until then, a 250-foot tower at Wallops Island had been the highest used in sonic boom testing.) During the summer and fall of 1970, the FRC’s F-104s from Edwards made 121 boom-generating flights to provide measurements of several still poorly understood aspects of the sonic boom, especially the places, known mathematically as caustics, where nonlinear focusing of acoustical rays occurs. Frequently caused by speeds very near Mach 1 or by acceleration, they can result in U-shaped signatures with bow and tail wave overpressures strong enough to create super booms. The BREN Tower allowed such measurements to be made in the vertical dimension for the first time. This test resulted in definitive data on the formation and nature of caustics, information that would be valuable in helping pilots to avoid making focused booms.[385]
For all intents and purposes, the results of earlier testing and surveys had already helped to seal the fate of the SST before the reports on this latest test began coming in. Yet the data gathered from 1958 through 1970 during the SST program contributed tremendously to the international aeronautical and scientific communities’ understanding of one of the most baffling and complicated aspects of supersonic flight. As Harry Carlson told the Nation’s top sonic boom scientists and engineers on the very same day of the last F-104 mission over Jackass Flats: "The importance of flight-test programs cannot be overemphasized. These tests have provided an impressive amount of high-quality data, which has been of great value in the verification of theoretical methods for the prediction of nominal overpressures and in the estimation from a statistical standpoint of the modifying influence of unpredictable atmospheric nonuniformities.”[386]