At the beginning of this book we encountered the Greek philosophers who let their imaginations roam beyond the visible, everyday world. One was Democritus, who was forty years younger than Anaxagoras; apparently, they knew each other. Democritus was known as the “laughing philosopher” for his habit of seeing the lighter side of life and mocking human frailties. He developed an original idea of Leucippus into the atomic theory. According to Democritus, the physical world was made of microscopic, indivisible entities called atoms.1 The atoms were in constant motion and they could take up an infinite number of different arrangements; sensory attributes like hot, smooth, bitter, and acrid emerged from assemblages of atoms but were not properties of the atoms themselves.2 It’s a strikingly modern view of matter.3 By analogy, a beach looks smooth from a distance, but close up we see it’s actually composed of particles ranging from tiny sand grains to pebbles and boulders.
Democritus speculated in a similar way about the Milky Way, where its smoothness conceals the fact that it is composed of the combined light of myriad stars. Several centuries later, Archytas made a logical argument that there was space beyond the whirling crystalline spheres.4 Arriving at the edge of heaven, he imagined, and extending your arm or a staff, either it meets resistance and a physical boundary, or it extends beyond the edge. If the universe is contained, it must be contained within something larger. If we
can reach beyond the edge, we define a new edge and that must continue without limit. In this way he argued that space must be infinite.5 The Greek imagination leapt downward to the invisibly small and upward to the unknowably large. By the late twentieth century, scientists knew that the span of scales from the size of a proton to the size of the observable universe was 42 powers of ten.
Human imagination, however, is not so neatly bounded. We can easily imagine what is—a phenomenon that’s allowed by the laws of nature—as well as what isn’t—a phenomenon that violates laws of physics as we know them. There’s even a third, limbic category—a situation that doesn’t violate any laws of nature but which doesn’t occur in our universe.6 In the early twenty-first century, scientists are once again pushing the boundaries of physical explanation, small and large. So particle theorists dream of nine-dimensional vibrating strings deep within every subatomic particle—worlds within the world—and cosmologists dream of an ensemble of space-times with wildly different properties, of which our universe is just one example—worlds beyond the world.
On Earth, the horizon is the farthest you can see because of the curvature of the surface of the planet. The distance is surprisingly small; standing in a small boat on a large body of water you could only see three miles in any direction. Sailors in antiquity were familiar with the slow disappearance of a ship as it sailed away, until only the tip of the mast was visible.7 This led them to speculate and search for exotic lands that might lie “beyond the horizon.” Astronomy also has the concept of a horizon as a limit to our view or to our knowledge. The event horizon emerges from the general theory of relativity. Einstein’s formulation of gravity is geometric; instead of the linear and absolute space and time of Newton’s theory, Einstein made an explicit connection between space and time and posited that mass curves space. A compact enough object will distort space-time sufficiently to form a black hole and the event horizon is the surface that seals a black hole off from the rest of the universe.8 It’s not a physical barrier. Rather, it’s an information membrane; radiation and matter can pass inward but not outward. Black holes are enigmatic because the region inside the event horizon lies beyond the scrutiny of observations. Cosmology has its own version of this idea, which is complicated by the expansion of the universe. The “particle” horizon for the universe divides events into those we can or can’t see at the present time. The universe had an origin, so there are regions from which light has not had time to reach us in the 13.8 billion years since the big bang. If we’re patient, light from ever more distant regions will reach us, so the observable universe grows with time. Meanwhile, the “event” horizon of the universe is the boundary between events that are visible at some time or another and events that aren’t visible at any time.9 Standard big bang cosmology predicts the existence of space-time beyond our horizon, possibly containing many star systems in addition to the 1023 visible through our telescopes. There are many worlds beyond view, perhaps more than we can imagine.
IIIII
Observational astronomy is a young activity. After tens of thousands of years of naked-eye observing, the telescope is just four hundred years old. Space astronomy is only fifty years into development, and the cost of launching a big glass into orbit means that the Hubble Space Telescope isn’t even among the fifty largest optical telescopes.10 In what follows we summarize what the future might hold for space science and astronomy, in terms of the missions recently launched or those on the near horizon, a few years from launch. The mid-horizon is five or ten years from now, where the uncertain funding landscape and the high cost of missions make the view very indistinct. The far horizon can depend on technologies not yet developed or perfected and is beyond view. Any projection of more than a few decades is in the realm of speculation and imagination. To close this book, we consider three pairs of missions that promise to advance our knowledge of other worlds—two within the Solar System, two looking at nearby stellar systems, and two studying the distant universe.
The heir to Viking and the Mars Exploration Rovers is the Mars Science Laboratory, or MSL.11 Following the tradition they established with Spirit and Opportunity, NASA asked students to submit essays to give MSL a name. The winner, from among over nine thousand entries, was sixth grader Clara Ma, who wrote: “Curiosity is an everlasting flame that burns in everyone’s mind. It makes me get out of bed in the morning and wonder what surprises life will throw at me that day.”12 And so the most sophisticated robot ever built was named Curiosity. Clara Ma got to inscribe her name on the metallic skin of the rover before it was bundled up for launch. Over a million people worldwide are part of the mission in a smaller way, by having their names added digitally to a microchip onboard. The public is clearly engaged in this Mars rover. In the months leading up to launch, Curiosity had over 30,000 followers on Twitter (plate 23).
The Mars Science Laboratory is a mission with a high degree of difficulty. Technical problems forced it to slip from a launch window in 2009 to the next one in late 2011, and meanwhile the budget ballooned to over $2.5 billion by the time of launch on November 26, 2011. Choosing a single place to land on Mars and answer profound questions about the entire planet was difficult; mission planners had a series of five workshops to whittle sixty possible sites down to four and they let the decision float as long as they could until selecting Gale Crater. It’s like playing roulette and “betting the farm” on a single number and a single spin of the wheel. The stakes are enormous—imagine trying to identify a single place on Earth that would be representative of all terrestrial geology and biology. Site selection was made a lot easier by maps from the Mars Reconnaissance Orbiter that shows features and surface rocks as small as a sofa. It hinged on engineering and safety constraints like having a navigable terrain and avoiding high latitudes where less energy is available to run the rover. Beyond that, the key landing site requirement was habitability: evidence for presence of surface water in the past.13 It’s hoped that Gale Crater will show evidence of once having been a shallow lake bed.
Pathfinder, which crawled over Mars in 1997, was little bigger than a child’s radio-controlled toy car. Spirit and Opportunity were the size of golf carts. Curiosity is like a small SUV. It’s ten feet long, nine feet wide, seven feet tall, and it weighs a ton.14 With that large mass, NASA couldn’t use the “bouncing airbag” landing method of previous rovers, where retro rockets slowed the lander and the payload sprouted airbags on all sides to protect it as it bounced to a lazy halt in the gentle Martian gravity. MSL used a procedure challenging enough to have flight engineers reaching for the Tums when the spacecraft started its final maneuvers 150 million miles from Earth with no real-time control possible. It steered through a series of S-shaped curves to lose speed, similar to those used by astronauts in landing the Space Shuttle. The target landing area is twelve miles across; that sounds large but it’s five times smaller than for any previous lander. A parachute slowed its descent for three minutes, then jettisoned its heat shield, leaving the rover exposed inside an aeroshell, attached to a “sky crane” mechanism. Retro rockets on the upper rim of the aeroshell further slowed the descent and then the aeroshell and parachute were jettisoned. At that point, the sky crane became the descent vehicle, with its own retro rockets guiding it toward the surface. About a hundred meters above the surface the sky crane slowed to a hover, and it lowered the rover to the surface on three cables and an electrical umbilical cord. The rover made a soft “wheels-down” landing and the connections were released (and severed as a fallback if the release mechanism were to fail). The sky crane flew off to crash land at a safe distance.15 Landing was set for August 6, 2012. The rover then readied itself for two years of exploring the red planet. Whew.
That was the plan. And the outcome: everything worked flawlessly. All the scary outcomes and potential disasters were avoided and the spacecraft touched down gently near the edge of Gale Crater on August 6, 2012. Hundreds of engineers cheered, and the millions who watched online shared the pride of the team in their technical feat. In fact, the landing worked so well that NASA has decided to use the technology again to launch another rover in 2020, with an entirely different set of scientific instruments onboard.
Curiosity has a science payload five times heavier than any previous Mars mission. There are ten instruments; eight have U. S. investigators, and one each comes from Russia and Spain. Curiosity has a titanium robotic arm with two joints at the shoulder, one at the elbow, and two at the wrist. The arm can extend seven feet from the rover, and it’s powerful enough to pulverize rocks while being delicate enough to drop an aspirin tablet into a thimble. The arm is versatile.16 It has a hand lens imager that can see details smaller than the width of a human hair, it has a spectrometer that can identify elements, it has a geologist’s rock brush, and it has tools for scooping, sieving, and delivering rock and soil samples to instruments within the rover. A set of three instruments called “Sample Analysis at Mars” will analyze the atmosphere and samples collected by the arm, with an emphasis on the detection of organic molecules and isotopes that trace the history of water in the rocks.17 The arm will deliver samples to another instrument that uses X-ray spectroscopy and fluorescence methods to analyze the complex mixture of minerals in a typical rock or sample of soil. Engineers took instruments that would fill a living room on Earth and squeezed them into a space the size of a microwave oven on Curiosity.
Film director James Cameron lobbied hard for a high-resolution 3D zoom camera to be included in the mission. The option for 3D imaging had been cut for budgetary reasons in 2007, but NASA was savvy enough to recognize a compelling public relations angle, so Cameron was appointed as a Mastcam co-investigator and informally as a “public engagement co-investigator” and the option was reinstated. However, in early 2011 the idea was shelved because there wasn’t enough time to fully test the cameras before launch.18 Curiosity lost the potential for cinematic 3D imaging with a director’s eye, but retained its fixed focal length cameras, which should return crisp 3D images for the duration of the mission. The mast cameras will view Mars from eye level, giving the public a sense of roaming on a distant world themselves. Nearly compensating for the loss of Cameron’s cinematography is a hi – tech instrument called ChemCam, which uses laser pulses to vaporize rocks at a distance of up to seven meters.19 It then performs a chemical analysis of the vapor, returning results within seconds. A “Star Wars” capability like that will undoubtedly capture the public imagination.
If Curiosity’s landing site is likened to a crime scene where damage was caused by the action of water, Curiosity is trying to identify the culprit long after they have left the scene. It will tell us beyond any reasonable doubt whether at least one part of this small, arid world was once wet and hospitable for life. Curiosity isn’t designed to find life. It’s not designed to detect DNA or other essential biological molecules, and it can’t look for fossils in the rocks it studies.20 Rather, it’s designed to detect organic compounds and provide an inventory of life’s essential elements—carbon, hydrogen, nitrogen, oxygen, sulfur, and phosphorus. Viking’s tantalizing verdict on life of “not proven” resonates among planetary scientists so they’re cautious in setting expectations for Curiosity, but the improvements will be dramatic. Unlike the Vikings, Curiosity will be at a site almost certain to have been wet in the past. It can roam widely for its samples; the Vikings could only gather soil their arms could reach. Curiosity will do analyses of the interiors of rocks and it will be extraordinarily sensitive, able to detect one part in a billion of organic compounds. It will test the hypothesis that perchlorate can mask the detection of organics. It will decide if low levels of Martian methane are more likely to be geological or biological in origin. It will even study the pattern of carbon atoms in any organic material it detects. Dominance of even or odd numbers of carbon atoms, as opposed to random mixtures of each, would be evidence of the repetitious subunits seen in biological assembly.21 Early results indicate that the Martian soil is chemically complex and potentially life-bearing.
And then we’ll have to wait. The middle horizon for Solar System exploration is cloudy.22 As impressive as Curiosity is, there’s only so much that can be learned from a small payload of instruments shipped to the Martian surface. If chunks of Mars could be brought back to Earth they could be analyzed in exhaustive detail, molecule by molecule. So sample return has long been the “Holy Grail” of Mars exploration.23 Unfortunately it will be difficult and expensive. The current plan is for two rovers to go to the same site on Mars and drill down as much as six feet for samples, then “cache” them in a sealed storage unit. Then a rocket would be required to boost the samples into Mars orbit, and finally a third mission would ferry them to Earth. Just the first part of this procedure will cost at least $2.5 billion and we wouldn’t have the samples in our hands until 2022 or even later.
The alternative is equally exciting: a study of “water worlds” in the outer Solar System. NASA and ESA combined forces to draw up plans for a flagship mission to interesting targets far from the Earth. There were two concepts: an orbiter to study the Jovian moons Europa and Ganymede and another to study Saturn’s moon Titan. After a “shootout” in 2009, it was announced that the Jupiter mission would go first. The Europa Jupiter System Mission,24 or Laplace, will launch no earlier than 2020 and would reach the Jovian system in 2028, and it will consist of two orbiters: one built by NASA to study Europa and Io and another built by ESA to study Ganymede and Callisto. Just NASA’s part of this project has a price tag of $4.7 billion so funding is not yet guaranteed.25 If all goes well, 420 years after Galileo first noted Jupiter’s largest moons, they will be observed in unprecedented detail by robotic spacecraft.
These missions could cement the idea that “habitable worlds” don’t just include Earth-like planets, but also large moons of giant planets. Mars lies beyond the edge of the traditional habitable zone, where water can be liquid on the surface of a planet. The outer Solar System planets are miniature versions of the Sun in chemical composition, and so uninteresting for potential biology. However, large moons like Europa and Ganymede almost certainly have watery oceans under crusts of ice and rock. Tidal and geological heating provide a localized energy source so all the ingredients for biology are there. NASA’s orbiter will measure threedimensional distribution of ice and water on Europa and determine whether the icy crust has compounds relevant to prebiotic chemistry.26 ESA’s orbiter will do the same for the enigmatic moon Ganymede, the largest in the Solar System.27 The biological “real estate” of the cosmos could easily be dominated by moons like these, languishing far from their stars’ warming rays.
The second pair of missions is exploring new worlds farther from home, across swaths of the Milky Way galaxy. When the first planet beyond the Solar System was first discovered in 1995, it marked the dramatic end of decades of searching and frustration. A planet like Jupiter reflects hundreds of millions of times less light than is emitted by its parent star, and from afar it will appear very close to the star, like a firefly lost in a floodlight’s glare. Astronomers therefore used stealth to discover exoplanets. They monitored the spectra of stars like the Sun and looked for Doppler shifts in the spectra caused by giant planets tugging on the star.28 This method has been an unqualified success; the number of exoplanets doubles every eighteen months, and more than three thousand have been discovered.29 It’s a major step in the continu-
ing Copernican Revolution—planets form throughout the galaxy as a natural consequence of star formation.
Timing is everything in science. It’s often fruitless to be too far ahead of your time, but being slow to innovate means being left in the dust. Bill Borucki hit the sweet spot with his idea of a different way to find exoplanets. Working at NASA Ames Research Center, he realized that a planet transiting across its star would dim it slightly, by an amount equal to the ratio of the area of the planet to the area of the star. It was a statistical method since even if all stars had planets, only a small fraction of them would be oriented so that the planet passed in front of the star. He realized that with the stability of the space environment, it would be possible to not only detect the 1 percent dimming caused by a Jupiter transit but to detect the 0.01 percent dimming of an Earth transit (figure 13.1). This was the chance to find other worlds like ours in distant space.30
Borucki and his team pitched the project to NASA Headquarters in 1992, but it was rejected as being technically too difficult. In 1994 they tried again, but this time it was rejected as being too expensive. In 1996, and then again in 1998, the proposal was rejected on technical grounds, even though lab work had been done
on the proof of concept. By the time the project was finally given the go ahead as a NASA Discovery class mission in 2001, exoplanets had been discovered and the first transits had been detected from the ground.31 Persistence had paid off.
Kepler was launched in March 2009 and almost immediately showed it had the sensitivity to detect Earths. It is using a one- meter mirror to “stare” at about 150,000 stars in the direction of the Cygnus constellation. Designed to gather data for 3.5 years, its mission was extended in early 2012 through 2016, which is long enough for it to succeed in its core mission: conducting a census of Earth-like planets transiting Sun-like stars. Early in 2013, the Kepler team announced results from the first two years of data. The results were spectacular: over 2,700 high-probability candidates (plate 24), more than quadrupling the number of known exoplanets.32 Nearly 350 of these were similar to the Earth in size, and fifty-four were in the habitable zones of their stars, ten of which were Earth-sized. The team projected that 15 percent of stars would host Earths and 43 percent would host multiple planets. The estimated number of habitable worlds in the Milky Way is a hundred million. The imagination struggles to contemplate what might exist on so many potentially living worlds.
Kepler is looking at stars within a few hundred light-years, our celestial backyard. Meanwhile, astronomers have their sights set on mapping larger swaths of our stellar system. Due for a launch by the European Space Agency in 2013, Gaia is the heir to Hip – parcos, measuring all stars in the Milky Way down to a level a million times fainter than the naked eye can see. The heart of Gaia is a pair of telescopes and a two-ton optical camera, feeding a focal plane tiled with over a hundred electronic detectors. Kepler has a camera with 100 million pixels; Gaia’s camera has a billion.33 Hipparcos studied 100,000 stars; Gaia will chart the positions, colors, and brightness levels of a billion stars. Not only that, it will measure each star a hundred times, for a total of a hundred billion observations. Like WMAP, Gaia will be at the L2 Lagrange point a million miles from Earth, gently spinning to scan the sky during its five-year mission.34 The result will be a stereoscopic 3D map of our corner of the Milky Way galaxy.
Gaia will detect planets, although that’s not its primary objective. It will carry out a full census of Jupiters within 150 light – years, not by looking for a Doppler shift or an eclipse of the parent star but by actually seeing the star wobble as it is tugged to and fro by the planet. This is a truly minuscule effect—the Sun wobbles around its edge due to the influence of Jupiter, but Gaia will be looking for a similar motion on stars hundreds of trillions of miles away. It’s like trying to see a penny pirouetting about its edge on the surface of the Moon. As many as 50,000 exoplanets may be discovered this way, compared to the current census of a few thousand candidates. Gaia will also detect tens of thousands of dark and warm worlds called brown dwarfs, which represent the “twilight” state of stars not massive enough to initiate nuclear fusion. It will also be able to detect dwarf planets like Pluto in the remote reaches of the Solar System. Such planets are distinctive and interesting geological worlds in their own right.
Gaia represents our “coming of age” in the Milky Way. It also provides a bridge to the last pair of missions we will consider, since the motions of the oldest stars in our galaxy were imparted at birth, so those motions represent a “frozen record” of how the galaxy formed. Current theories suggest that large galaxies were assembled from the mergers of smaller galaxies. Most of these mergers occurred billions of years ago, but traces remain in the form of streams of stars threading the halo of the galaxy like strands of spaghetti.35 This type of galactic archaeology is a vital part of understanding how the universe turned from a smooth and hot gas to a cold void sprinkled with “island universes” of stars.
Cosmology is a vibrant field, spurred by new observational capabilities, computer simulations, and increasingly tried-and-tested theories of gravity and the big bang origin of the universe. The mid-horizon in cosmology is defined by the James Webb Space Telescope (JWST), which is an ambitious successor to the Hubble Space Telescope.36 Hubble’s mirror is 2.4 meters in diameter, limited by the size of payload the Space Shuttle could heft into a low Earth orbit. JWST will gather seven times more light with its 6.5-meter mirror, even though it will be launched on an Ariane 5 rocket, which has a maximum payload diameter of 4 meters
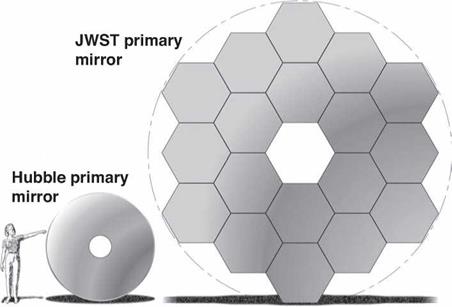
Figure 13.2. The James Webb Space Telescope is the successor to the Hubble Space Telescope, due for launch in 2018. It will be located a million miles from the Earth and will unfold in orbit to become the largest telescope ever deployed in space. Its primary goal is to detect “first light” in the universe, when the first stars and galaxies formed, over 13 billion years ago (NASA/JWST).
|
(figure 13.2). This clever trick is accomplished by a design where the segments of the telescope unfold after it is deployed, like flower petals, adding greatly to the engineering challenge. JWST will also be located at L2, the increasingly crowded “watering hole” of many astrophysics missions. The telescope and its instruments will be too far away to be serviced by astronauts, so the stakes are high and everyone involved in the project feels the pressure. JWST’s cost has ballooned to over $8 billion and the launch date has slipped several times, with a current estimate of 2018. Although the project has broad support from the astronomical community, the high cost and repeated delays have brought it close to cancellation. NASA can only afford one flagship planetary science mission like MSL at a time, and it can only afford one flagship astronomy mission at a time. Until JWST is operating, no other big concept can get traction and a funding start. Astronomers struggle to maintain solidarity under such conditions.
Since JWST is a general-purpose telescope, it will contribute to the study of exoplanets as well as the more remote universe. One of its instruments can take spectra of the feeble light reflected by a planet next to a remote star. By observing in the infrared, the exoplanet will be ten to twenty times brighter relative to the star than it would be at visible wavelengths. Astronomers will take the most Earth-l ike planets and search for spectral features due to oxygen and ozone. These two gases are telltale indicators of life on Earth, and if they’re seen in abundance in the atmospheres of exoplanets, it will be evidence of photosynthetic organisms elsewhere. The observations are extremely challenging, requiring a hundred hours or more per target.37 But if they succeed, the detection of biomarkers will be a dramatic step in our quest to find a world like our own.
However, the core mission of JWST is to detect “first light.” First light is the time in the youthful universe when gravity has had time to congeal the first structures from the rapidly expanding and cooling gas. The big bang was unimaginably hot—a cauldron intense enough to melt the four forces of nature into one super-force. By 400,000 years after the big bang, the temperature had dropped to 3000 K and the universe was glowing dull red as the fog lifted and the photons of the radiation from the big bang began to travel freely through space. The “Dark Ages” began.38 Theorists are not sure of the exact timing, but they think that it took another hundred million years or so for small variations in density to grow enough for pockets of gas to collapse. This would have been when the universe was at room temperature, a hundred times smaller, and a million times denser than it is today. At this point the Dark Ages ended. It’s likely that stars formed first and went through a number of cycles of birth and death before they agglomerated into galaxies. The first stars were probably massive, 80-100 times the mass of the Sun, and they died quickly and violently, leaving behind black holes. In this strange universe no worlds like the Earth existed. The universe was made of hydrogen and helium; it would take generations of stars to live and die before enough heavy elements were created for planets and biology to be possible.
Even JWST will probably not be able to detect the first stars, but it should be able to detect the first galaxies. Its superior capabilities are required because the Hubble Space Telescope has almost exhausted its light grasp as it looks for the first light. Less than 500 million years after the big bang, light travels so far and for so long to reach us that cosmic expansion stretches visible photons to near infrared wavelengths. Distant light slides off the red end of the spectrum and becomes invisible. In a sense, JWST is really looking for “first heat.”
To take us beyond the horizon, we look to a mission that is currently taking data. Planck is the successor to WMAP, launched by the European Space Agency in 2009. It has a primary mirror about the size of a dining room table made of carbon fiber reinforced plastic coated with a thin layer of aluminum, weighing only twenty-eight pounds. The detectors are cooled to just above absolute zero. Planck improves on WMAP by a factor of three in angular resolution, a factor of ten in wavelength coverage, and a factor of ten in sensitivity. All of this means Planck will extract fifteen times more information from the cosmic microwave background than WMAP. Nobody doubts the big bang anymore, so Planck is taking the theory to its limits, testing inflation, the notion that the universe underwent a phase of exponential expansion an amazing 10-35 seconds after the big bang.39 By making measurements with a precision of a millionth of a degree, the Planck mission may be able to determine how long inflation lasted and detect the signature of primordial gravitational waves imprinted on the microwaves. Or it may not provide any support for inflation, driving scientists back to the drawing board.
If inflation occurred, quantum fluctuations from the dawn of time became the seeds for galaxy formation. If inflation occurred, there are regions of space and time far beyond the reach of our best telescopes. If inflation occurred, one quantum fluctuation grew to become the cold and old universe around us. The initial conditions of the big bang can accommodate many quantum fluctuations, each of which gives rise to a universe with randomly different physical properties. In most of those universes the properties would be unlikely to support long-lived stars and the formation of heavy elements and life. Inflation therefore supports the “multi – verse” concept, where there are myriad other universes with different properties, unobservable by us, and we happen to live in one of the rare universes hospitable for life and intelligent observers.40
In fact, the multiverse offers so many possibilities that everything that could possibly happen actually will happen somewhere. There might not just be clones of Earth beyond the horizon, but clones of us as well. Quantum genesis and the multiverse take us to a point where science becomes as wild and bold as any science fiction—a vision of worlds without end.
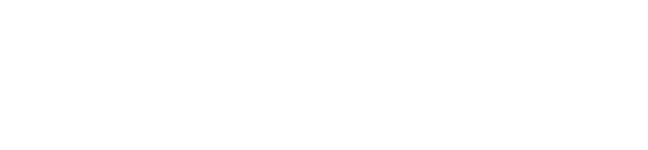