If composites were to receive wide application, the cost of the materials would have to dramatically decline from their mid-1980s levels. ACEE succeeded in making plastic composites commonplace not just in fairings and hatches for large airliners but also on control surfaces, such as the ailerons, flaps, and rudder. On these secondary structures, cash – strapped airlines achieved the weight savings that prompted the shift to composites in the first place. The program did not, however, result in the immediate transition to widespread production of plastic composites for primary structures. Until the industry could make that transition, it would be impossible to justify the investment required to create the infrastructure that Lovelace described to produce composites at rates equivalent to yearly aluminum output.
To the contrary, tooling costs for composites remained high, as did the labor costs required to fabricate the composite parts.[748] A major issue driving costs up under the ACEE program was the need to improve the damage tolerance of the composite parts, especially as the program transitioned from secondary components to heavily loaded primary structures. Composite plastics were still easy to damage and costly to replace. McDonnell-Douglas once calculated that the MD-11 trijet contained about 14,000 pounds of composite structure, which the company estimated saved airlines about $44,000 in yearly fuel costs per plane.[749] But a single incident of "ramp rash” requiring the airline to replace one of the plastic components could wipe away the yearly return on investment provided by all 14,000 pounds of composite structure.[750]
The method that manufacturers devised in the early 1980s involved using toughened resins, but these required more intensive labor to fabricate, which aggravated the cost problem.[751] From the early 1980s, NASA
worked to solve this dilemma by investigation new manufacturing methods. One research program sponsored by the Agency considered whether textile-reinforced composites could be a cost-effective way to build damage-tolerant primary structures for aircraft.[752] Composite laminates are not strong so much as they are stiff, particularly in the direction of the aligned fibers. Loads coming from different directions have a tendency to damage the structure unless it is properly reinforced, usually in the form of increased thickness or other supports. Another poor characteristic of laminated composites is how the material reacts to damage. Instead of buckling like aluminum, which helps absorb some of the energy caused by the impact, the stiff composite material tends to shatter.
Some feared that such materials could prove too much for cash- strapped airlines of the early 1990s to accept. If laminated composites were the problem, some believed the solution was to continue investigating textile composites. That meant shifting to a new process in which carbon fibers could be stitched or woven into place, then infused with a plastic resin matrix. This method seemed to offer the opportunity to solve both the damage tolerance and the manufacturing problems simultaneously. Textile fibers could be woven in a manner that made the material strong against loads coming from several directions, not just one. Moreover, some envisioned the deployment of giant textile composite sewing machines to mass-produce the stronger material, dramatically lowering the cost of manufacture in a single stroke.
The reality, of course, would prove far more complex and challenging than the visionaries of textile composites had imagined. To be sure, the concept faced many skeptics within the conservative aerospace industry even as it gained force in the early 1990s. Indeed, there have been many false starts in the composite business. The Aerospace America journal in 1990 proposed that thermoplastics, a comparatively little – used form of composites, could soon eclipse thermoset composites to become the "material of the ’90s.” The article wisely contained a cautionary note from a wry Lockheed executive, who recalled a quote by a former boss in the structures business: "The first thing I hear about a new material is the best thing I ever hear about it. Then reality sinks in, and it’s a matter of slow and steady improvements until you achieve the properties you want.”[753] The visionaries of textile composite in the late
1980s could not foresee it, but they would contend with more than the normal challenges of introducing any technology for widespread production. A series of industry forces were about to transform the competitive landscape of the aerospace industry over the next decade, with a wave of mergers wreaking particular havoc on NASA’s best-laid plans.
It was in this environment when NASA began the plunge into developing ever-more-advanced forms of composites. The timeframe came in the immediate aftermath of the ACEE program’s demise. In 1988, the Agency launched an ambitious effort called the Advanced Composites Technology (ACT) program. It was aimed at developing hardware for composite wing and fuselage structures. The goals were to reduce structural weight for large commercial aircraft by 30-50 percent and reduce acquisition costs by 20-25 percent.[754] NASA awarded 15 contracts under the ACT banner a year later, signing up teams of large original equipment manufacturers, universities, and composite materials suppliers to work together to build an all-composite fuselage mated to an allcomposite wing by the end of the century.[755]
During Phase A, from 1989 to 1991, the program focused on manufacturing technologies and structural concepts, with stitched textile preform and automated tow placement identified as the most promising new production methods.[756] "At that point in time, textile reinforced composites moved from being a laboratory curiosity to large scale aircraft hardware development,” a NASA researcher noted.[757] Phase B, from 1992 to 1995, focused on testing subscale components.
Within the ACT banner, NASA sponsored projects of wide-ranging scope and significance. Sikorsky, for example, which was selected after 1991 to lead development and production of the RAH-66 Comanche, worked on a new process using flowable silicone powder to simplify the process of vacuum-bagging composites before being heated in an autoclave.[758] Meanwhile, McDonnell-Douglas Helicopter investigated 3-D
finite element models to discover how combined loads create stresses through the thickness of composite parts during the design process.
The focus of ACT, however, would be aimed at developing the technologies that would finally commercialize composites for heavily loaded structures. The three major commercial airliner firms that dominated activity under the ACEE remained active in the new program despite huge changes in the commercial landscape.
Lockheed already had decided not to build any more commercial airliners after ceasing production of the L-1011 Tristar in 1984 but pursued ACT contracts to support a new strategy—also later dropped—to become a structures supplier for the commercial market.[759] Lockheed’s role involved evaluating textile composite preforms for a wide variety of applications on aircraft.
It was still 8 years before Boeing and McDonnell-Douglas agreed to their fateful merge in 1997, but ACT set each on a path for developing new composites that would converge around the same time as their corporate identities. NASA set Douglas engineers to work on producing an all-composite wing. Part of Boeing’s role under ACT involved constructing several massive components, such as a composite fuselage barrel; a window belt, introducing the complexity of material cutouts; and a full wing box, allowing a position to mate the Douglas wing and the Boeing fuselage. As ambitious as this roughly 10-year plan was, it did not overpromise. NASA did not intend to validate the airworthiness of the technologies. That role would be assigned to industry, as a private investment. Rather, the ACT program sought to merely prove that such structures could be built and that the materials were sound in their manufactured configuration. Thus, pressure tests would be performed on the completed structures to verify the analytical predictions of engineers.
Such aims presupposed some level of intense collaboration between the two future partners, Boeing and McDonnell-Douglas, but NASA may have been disappointed about the results before the merger of 1997. Although the former ACEE program had achieved a level of unique collaboration between the highly competitive commercial aircraft prime contractors, that spirit appeared to have eroded under the intense market pressures of the early 1990s airline industry. One unnamed industry source explained to an Aerospace Daily reporter in 1994: "Each company
wants to do its own work. McDonnell doesn’t want to put its [composite] wing on a Boeing [composite] fuselage and Boeing doesn’t trust its composite fuselage mated to a McDonnell composite wing.”[760]
NASA, facing funding shortages after 1993, ultimately scaled back the goal of ACT to mating an all-composite wing made by either McDonnell- Douglas or Boeing to an "advanced aluminum” fuselage section.[761] Boeing’s work on completing an all-composite fuselage would continue, but it would transition to a private investment, leveraging the extensive experiences provided by the NASA and military composite development programs.
In 1995, McDonnell-Douglas was selected to enter Phase C of the ACT program with the goal to construct the all-composite wing, but industry developments intervened. After McDonnell-Douglas was absorbed into Boeing’s brand, speculation swirled about the fate of the former’s active all-composite wing program. In 1997, McDonnell-Douglas had plans to eventually incorporate the new wing technology on the legacy MD-90 narrow body.[762] (Boeing later renamed MD-90 by filling a gap created when the manufacturer skipped from the 707 to the 727 airliners, having internally designated the U. S. Air Force KC-135 refueler the 717.[763] [764]) One postmerger speculative report suggested that Boeing might even consider adopting McDonnell-Douglas’s all-composite wing for the Next Generation 737 or a future variant of the 757. Boeing, however, would eventually drop the all-composite wing concept, even closing 717 production in 2006.
The ACT program produced an impressive legacy of innovation. Amid the drive under ACT to finally build full-scale hardware, NASA also pushed industry to radically depart from building composite structures through the laborious process of laying up laminates. This process not only drove up costs by requiring exorbitant touch labor; it also produced material that was easy to damage without adding bulk—and weight—to the structure in the form of thicker laminates and extra stiffeners and doublers.
The ACT formed three teams that combined one major airframer each, with several firms that represented part of a growing and
increasingly sophisticated network of composite materials suppliers to the aerospace industry. A Boeing/Hercules team focused on a promising new method called automated tow placement. McDonnell-Douglas was paired with Dow Chemical to develop a process that could stitch the fibers roughly into the shape of the finished parts, then introduce the resin matrix through the resin transfer molding (RTM) process.123 That process is known as "stitched/RTM.”[765] Lockheed, meanwhile, was tasked with BASF Structural Materials to work on textile preforms.
NASA and the ACT contractors had turned to textiles full bore to both reduce manufacturing costs and enhance performance. Preimpregnating fibers aligned unidirectionally into layers of laminate laid up by hand and cured in an autoclave had been the predominant production method throughout the 1980s. However, layers arranged in this manner have a tendency to delaminate when damaged.[766] The solution proposed under the ACT program was to develop a method to sew or weave the composites three-dimensionally roughly into their final configuration, then infuse the "preform” mold with resin through resin transfer molding or vacuum-assisted resin transfer molding.[767] It would require the invention of a giant sewing machine large and flexible enough to stitch a carbon fabric as large as an MD-90 wing.
McDonnell-Douglas began the process with the goal of building a wing stub box test article measuring 8 feet by 12 feet. Pathe Technologies, Inc., built a single-needle sewing machine. Its sewing head was computer controlled and could move by a gantry-type mechanism in the x – and y-axes to sew materials up to 1 inch in thickness. The machine stitched prefabricated stringers and intercostal clips to the wing skins.[768] The wings skins had been prestitched using a separate multineedle machine.[769] Both belonged to a first generation of sewing machines that accomplished their purpose, which was to provide valuable data and experience. The single-needle head, however, would prove far too limited. It moved only 90 degrees in the vertical and horizontal planes,
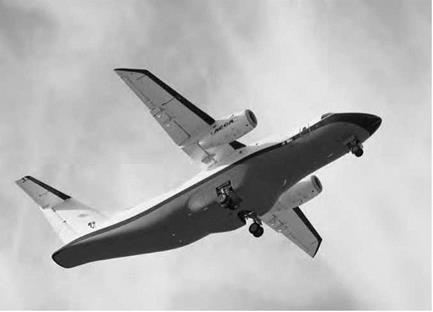
The Advanced Composite Cargo Aircraft is a modified Dornier 328Jet aircraft. The fuselage aft of the crew station and the vertical tail were removed and replaced with new structural designs made of advanced composite materials fabricated using out-of-autoclave curing. It was developed by the Air Force Research Laboratory and Lockheed Martin. Lockheed Martin.
|
meaning it was limited to stitching only panels with a flat outer mold line. The machine also could not stitch materials deeply enough to meet the requirement for a full-scale wing.129
NASA and McDonnell-Douglas recognized that a high-speed multineedle machine, combined with an improved process for multiaxial warp knitting, would achieve affordable full-scale wing structures. This so-called advanced stitching machine would have to handle "cover panel preforms that were 3.0m wide by 15.2m long by 38.1mm thick at speeds up to 800 stitches per minute. The multiaxial warp knitting machine had to be capable of producing 2.5m wide carbon fabric with an areal weight of 1,425g/m2.”130 Multiaxial warp knitting automates the process of producing multilayer broad goods. NASA and Boeing selected the resin film infusion (RFI) process to develop a wing cost-effectively.
Boeing’s advanced stitching machine remains in use today, quietly producing landing gear doors for the C-17 airlifter. The thrust of [770] [771]
innovation in composite manufacturing technology, however, has shifted to other places. Lockheed’s ACCA program spotlighted the emergence of a third generation of out-of-autoclave materials. Small civil aircraft had been fashioned out of previous generations of this type of material, but it was not nearly strong enough to support loads required for larger aircraft such as, of course, a 328Jet. In the future, manufacturers hope to build all-composite aircraft on a conventional production line, with localized ovens to cure specific parts. Parts or sections will no longer need to be diverted to cure several hours inside an autoclave to obtain their strength properties. Lockheed’s move with the X-55 ACCA jet represents a critical first attempt, but others are likely to soon follow. For its part, Boeing has revealed two major leaps in composite technology development on the military side, from the revelation of the 1990s-era Bird of Prey demonstrator, which included a single-piece composite structure, to the co-bonded, all-composite wing section for the X-45C demonstrator (now revived and expected to resume flight-testing as the Phantom Ray).
The key features of new out-of-autoclave materials are measured by curing temperature and a statistic vital for determining crashworthiness called compression after impact strength. Third-generation resins now making an appearance in both Lockheed and Boeing demonstration programs represent major leaps in both categories. In terms of raw strength, Boeing states that third-generation materials can resist impact loads up to 25,000 pounds per square inch (psi), compared to 18,000 psi for the previous generation. That remains below the FAA standard for measuring crashworthiness of large commercial aircraft but may fit the standard for a new generation of military cargo aircraft that will eventually replace the C-130 and C-17 after 2020. In September 2009, the U. S. Air Force awarded Boeing a nearly $10-million contract to demonstrate such a nonautoclave manufacturing technology.